Chapter 43 Molecular Imaging Approaches for Evaluation of Myocardial Pathophysiology
Angiogenesis, Ventricular Remodeling, Inflammation, and Cell Death
INTRODUCTION
The application of imaging using biologically targeted markers (i.e., molecular imaging) has a number of requirements.1 A molecular target that adequately represents the process being studied is critical to the specificity of any imaging approach. The target must then lend itself to a readily synthesizable probe(s) that will bind to the target molecule with a high degree of specificity. Lastly, an imaging technology that provides the best combination of sensitivity and resolution (both spatial and temporal) to identify and localize the probe within the target organ system needs to be immediately available and economically feasible. Molecular imaging approaches are currently being developed for most of the imaging modalities, including nuclear, magnetic resonance, x-ray computed tomography (CT), optical fluorescence, bioluminescence, and ultrasound.2 Though each modality carries strengths and weaknesses, it is likely that the practical limitations of cost and widespread availability will determine which modalities become adapted for clinical use.
Single-photon emission computed tomography (SPECT) and positron emission tomography (PET) are imaging techniques that make use of radiolabeled probes and have been used for over 3 decades. Radiolabeling has the unique advantage of augmenting low signal-intensity objects. For example, PET can detect picomolar and nanomolar concentrations of a molecule of interest.2 Though SPECT offers the advantage of decreased cost and widespread availability, PET offers the advantages of increased sensitivity in conjunction with the ability to quantitate as well as repetitively image using tracers with ultrashort half-lives. In the past, nuclear imaging modalities have been limited by attenuation artifacts from soft tissue and partial volume effects. More recent systems combining CT imaging with either SPECT or PET have allowed for attenuation correction, leading to improved imaging quantification and registration.
ANGIOGENESIS
Atherosclerosis is a chronic inflammatory disease that leads to the progression of lipid-laden vascular plaques.3,4 Monocyte adhesion and transendothelial migration result in activation and differentiation, with amplifying production of cytokines/chemokines, as well as foam cell development when these subendothelial macrophages endocytose oxidized lipid. The progression of atherosclerotic disease can lead to the development of chronic ischemia in areas of myocardium, secondary to poor perfusion through narrowed blood vessels. Subsequent signals in response to the ischemia may stimulate angiogenic and arteriogenic responses to restore perfusion.
Angiogenesis is defined as the process of sprouting new capillaries from preexisting microvessels.5 There is a great deal of interest in understanding the processes of angiogenesis in order to design therapeutic treatments that allow revascularization through an iatrogenic-stimulated angiogenic response. This angiogenic process often occurs in association with arteriogenesis, which represents a remodeling of larger preexisting vascular channels or collateral vessels feeding the new microvascular network. The goal for any myocardial revascularization strategy would be to initiate angiogenesis and arteriogenesis in manner that improves tissue perfusion. There is a large body of literature devoted to understanding these phenomena.6–8
Angiogenesis is stimulated by external processes such as ischemia, hypoxia, inflammation, and shear stress. Endothelial cells, smooth muscle cells, blood-derived macrophages, and circulating stem cells all play distinctive roles in the angiogenic process. There is the careful interaction of these cells with each other as well as within the tissue of extracellular matrix proteins. The process itself consists of a series of endothelial cell responses to angiogenic stimulation, such as degradation of extracellular matrix (ECM), budding from parent vessels, proliferation, migration, tube formation, and ultimately maturation and maintenance of the new vessel.9 Figure 43-1 outlines a schematic representation of the process of angiogenesis on an endothelial cellular level.
Hypoxia, the imbalance between oxygen delivery and demand in a given tissue, is a potent stimulator of angiogenesis.10 Hypoxic conditions such as myocardial ischemia from atherosclerotic disease or acute myocardial infarction (MI) result in up-regulation of the transcriptional activator hypoxia-inducible factor 1 (HIF-1).11 HIF-1 is a heterodimeric protein composed of alpha and beta subunits. It is continuously expressed and degraded through oxygen-dependent proline hydroxylation of the alpha subunit and subsequent ubiquitination by von Hippel-Lindau E3 ligase for degradation in the proteosome.12 Hypoxia stabilizes the HIF-1 protein by preventing the proline hydroxylation and ultimately the ubiquitination and degradation in the proteosome. This up-regulation of HIF-1 protein leads to the transcription of a number of hypoxia-inducible genes, including the key angiogenic mediators vascular endothelial growth factor (VEGF), platelet-derived growth factor (PDGF) and TGF-β, and the VEGF receptors, Flt-1 (VEGFR-1) and FLK-1 (VEGFR-2).10,13–16
When VEGF binds to its receptors on the surface of endothelial cells, a signal is transduced through their tyrosine kinase activity. This initiates a series of processes that results in endothelial cell proliferation, migration, survival, and angiogenesis.17 Early work in this field generated enthusiasm for the prospect of therapeutic angiogenesis utilizing angiogenic proteins like VEGF or fibroblast growth factor 2 (FGF-2). In fact, a number of trials had been designed to attempt therapeutic angiogenesis, with the goals of stimulating new blood vessel growth and improving myocardial perfusion in ischemic heart disease. With relatively invasive measures to assess efficacy of treatment, preclinical studies in animal models had shown some benefit to therapeutic angiogenesis, supporting the transition to human trials.18 Based on the early results, clinical trials were designed and initiated.
The FGF-2 Initiating Revascularization Support Trial (FIRST) randomized patients with chronic angina (class III-IV) to three doses of bFGF protein intracoronary injection versus placebo.19 The study demonstrated no significant differences between the groups in exercise time, nuclear perfusion, or quality of life at 90 days. The Angiogenic Gene Therapy Trial (AGENT) randomized patients to a single escalating intracoronary dose of replication-defective adenovirus containing the FGF4 gene.20 There was no significant difference in exercise treadmill time at 4 and 12 weeks.
In contrast, the VEGF in Ischemia for Vascular Angiogenesis (VIVA) Trial randomized patients with inducible ischemia on myocardial perfusion imaging to receive two (low- or high-dose) intracoronary injections of VEGF-1 protein versus placebo, followed by three more injections on days 3, 6, and 9.21 There were no differences in exercise time or anginal class between the groups at 60 days, but anginal class was improved at 120 days in the high-dose group. There were no differences in myocardial perfusion throughout all the groups. The Randomized Evaluation of VEGF for Angiogenesis in Severe Coronary Disease (REVASC) Trial involved intramyocardial injection of replication-defective adenovirus containing the VEGF121 gene.22 This study demonstrated a clinical improvement in the gene-therapy patients that was sustained from 3 to 6 months. Patients had significant improvements in exercise treadmill time to an additional 1 mm of ST depression, exercise time to angina, and angina class; however, nuclear perfusion imaging supported the control group, with the caveat that the treated group achieve higher workloads overall. More recently, the Euroinject One phase 2 clinical trial involved intramyocardial injections of plasmid containing VEGF in patients with Canadian Class 3 and 4 angina.23 The study revealed no differences in perfusion abnormalities, but there were improvements in regional ventricular wall-motion disturbances and functional anginal class.
One important signaling molecule is tie-2, a receptor tyrosine kinase, primarily expressed on vascular endothelium. Tie-2 has two major ligands: angiopoietin-1, which plays an agonistic signaling role, and angiopoietin-2, which appears to play an antagonistic signaling role.24,25 In conjunction with VEGF, this signaling mechanism appears to help stabilize and mature new capillaries as they develop, providing an important dimension to endothelial signaling and effective therapeutic angiogenesis. Preliminary, animal studies support utilizing this multipronged approach to VEGF angiogenesis therapy to obtain a more functional vasculature.26
Another significant signaling system is that of the Notch–Delta-like ligand pathway. The Notch receptor is a single-pass transmembrane protein consisting of an extracellular domain that is responsible for ligand interaction, a transmembrane domain that is involved in receptor activation, and an intracellular signaling domain.9 Signaling through Notch requires cell to cell contact through binding of its ligand, which is also a cell-surface protein. The ligands are members of the Jagged and Delta-like ligand family. Of interest to vascular endothelial cells are Notch 1 and Notch 4, as well as their ligands, Jagged1, Delta-like ligand 1 (Dll1), and Delta-like ligand 4 (Dll4). Dll4 is found exclusively on endothelial cells. Murine gene disruption experiments have revealed that Notch-Dll4 signaling is crucial to normal embryonic vascular development.27 Blocking the Notch-Dll4 interaction can paradoxically lead to an increase in angiogenesis and new vessel budding, but the new vessels appear to function in an abnormal manner, which compromises blood flow and oxygenation to the area of interest.28–30 It appears that VEGF up-regulates Dll4 in endothelial cells in a manner that creates a complex feedback loop to help achieve functional new blood vessels (Fig. 43-2). This argues for the importance of understanding what molecular switches are being activated in any attempt at therapeutic angiogenesis.
Endothelial signaling through VEGF and its concomitant receptor pathways is not the only molecular event undertaken during angiogenesis. There is also up-regulation and activation of molecules like integrins that aid in the cell-cell signaling and ultimately extracellular remodeling process. Integrins are a family of heterodimeric (αβ) cell-surface receptors that mediate divalent, cation-dependent, cell-cell, and cell-matrix adhesion and signaling through tightly regulated interactions with their respective ligands.31 During angiogenesis, endothelial cells make use of integrins to adhere to one another and the extracellular matrix to construct and extend new vessels. Peak expression of one integrin in particular, αvβ3 integrin, has been shown to occur 12 to 24 hours after initiation of angiogenesis with FGF2.32 Integrins are capable of mediating an array of cellular processes, including cell adhesion, migration, proliferation, differentiation, and survival via a number of signal transduction pathways (Fig. 43-3).33,34 Activation of c-Jun NH2-terminal kinase (JNK) and extracellular signal-regulated kinase (ERK) may lead to endothelial cell–induced remodeling of the ECM in response to mechanical stimuli. Specifically, the endothelial cell integrin αvβ3 allows cells to interact with the ECM in a way that aids in endothelial cell migration.35 Through outside-in signaling, integrin αvβ3 also plays a critical roll in the survival of cells undergoing angiogenesis.32
Several other molecules have been identified in this schema of endothelial cell activation. Syndecan-4 is a transmembrane heparan sulfate–carrying core protein that promotes binding of VEGF to VEGFR, resulting in activation of protein kinase C and downstream signaling.36 CD13 is a cell-surface antigen that is expressed in endothelial cells as an aminopeptidase—in other words, a membrane-bound metalloproteinase that appears to be essential for capillary tube formation.37 Degradation of the extracellular matrix involves up-regulation of matrix metalloproteinases, as well, to allow budding and expansion of the new vessel. Other molecular events include platelet-derived growth factor receptor signaling in response to HIF-1. This activates cell types like the perivascular pericytes or vascular smooth muscle cells, playing a crucial role in the new blood vessel development.38
RADIOTRACER-BASED IMAGING OF ANGIOGENESIS
Potential targets for molecular imaging of angiogenesis have been traditionally divided into three major categories: non-endothelial targets like molecules associated with monocytes, macrophages, and stem cells; endothelial cell targets like vascular endothelial growth factor (VEGF), integrins, CD13, and syndecan-4; and extracellular matrix proteins.39 Despite the wide array of molecules available as potential imaging targets for angiogenesis in response to ischemia, there are just two real active areas of research: imaging VEGF receptors via labeled VEGF and imaging the αvβ3 integrin via ligand-like analogs.
Vascular Endothelial Growth Factor Receptors
VEGF receptors have been targeted for imaging techniques in models of ischemia-induced angiogenesis. Radiolabeled-VEGF121 has been used to effectively identify angiogenesis in a rabbit model of hindlimb ischemia.40 In this study, KDR and Flt-1 receptor expression was increased in the immunohistochemistry analysis of the skeletal muscle, supporting the theoretical hypoxic-driven angiogenic response. Biodistribution of the radiotracer raises concern for the practical application of this system in humans. The biodistribution was 20-fold higher levels in critical organs (liver, kidneys) compared with ischemic limb and is presumably related to relative VEGFR density in these organ systems.
A PET tracer, copper-64-labeled (64Cu)-6DOTA-VEGF121, was recently developed for imaging angiogenesis in a rat model of MI (Fig. 43-4).41 Rats underwent ligation of the left coronary artery and subsequent PET imaging at various time points after MI. The investigators hypothesized that this tracer would detect early angiogenic signals because ischemia drives VEGFR expression. Co-registration of images was carried out using CT, and the zone of infarct was demonstrated using fluorine-18-labeled fluorodeoxyglucose (18F-FDG) uptake. The study demonstrated that 64Cu-6DOTA-VEGF121– specific signal was present in the infarct region and peaked on day 3, consistent with the changing levels of VEGFR expression in the tissue as analyzed by immunofluorescence microscopy. The same group has also published experiments supporting the use of 64Cu-6DOTA-VEGF121 for imaging of VEGFR-2 in a murine model of hindlimb ischemia-induced angiogenesis.42
Another type of cardiac-specific reporter has been developed as a gene expression system for use in rats, with microPET imaging (see Chapter 45 for specific details).43 Briefly, the system involves adenovirus delivery of mutated thymidine kinase under the control of a cytomegalovirus promoter driving expression in myocardial cells. The reporter probe is 18F-labeled fluoro-3-hydroxymethyl-butylguanine (18F-FHBG), which crosses the myocardial membrane and gets phosphorylated by the thymidine kinase. Phosphorylation essentially traps the 18F-FHGB in the myocardium for subsequent microPET imaging. Early studies revealed that the localized site of the mutated thymidine kinase, HSV1-sr39tk, corresponded closely with that defined by postmortem autoradiography, histology, and immunohistochemistry.44 Other studies have demonstrated the feasibility of utilizing a similar reporter system in pigs using a clinical PET scanner.45 The reporter system was then linked to VEGF to assess feasibility of developing an approach that links therapy and imaging.46 Early experiments with rat embryonic cardiomyocytes revealed a strong correlation in that both the mutated thymidine kinase and VEGF were expressed in the same cells. Further studies involved injection of the VEGF/thymidine kinase reporter system in models of ischemia. Using microPET, cardiac transgene expression was assessed and the in vivo imaging correlated well with ex vivo tissue studies for gamma counting, thymidine kinase activity, and VEGF levels. There appeared to be increased capillaries and small blood vessels in the VEGF-treated myocardium; however, there was no improvement in perfusion assessed by nitrogen-13 (13N)-ammonia imaging or metabolism assessed with 18F-FDG imaging. These studies suggest that a reporter system can be developed to help visualize the effectiveness of delivering VEGF gene therapies for stimulation of angiogenesis.
Integrin αvβ3
Imaging angiogenic vessels through targeting of αvβ3 integrin was first proposed through a series of magnetic resonance imaging studies using a monoclonal antibody to αvβ3 integrin tagged with a paramagnetic contrast agent.47 The studies were complicated by poor clearance of the tracer from the blood pool. Later studies made use of a number of αvβ3 antagonists that were radiolabeled.48,49 The experiments took advantage of the arginine-glycine-aspartate (RGD) binding sequence on integrins by synthesizing various RGD analogs.
An indium-111 (111In)-labeled quinolone (111In-RP748) revealed high affinity and selectivity for αvβ3 integrin in adhesion assays.50 Subsequent studies using a cy3-labeled homolog of 111In-RP748 demonstrated preferential binding to activated αvβ3 integrins on endothelial cells in culture, with localization to cell-cell contact points.51 Initial studies with this agent focused on imaging tumor angiogenesis, although the first imaging of ischemia-induced myocardial angiogenesis using 111In-RP748 was carried out in rat and canine models of infarction.52 In these studies, 111In-RP748 demonstrated favorable kinetics for in vivo SPECT imaging of ischemia-induced angiogenesis of the heart. Relative 111In-RP748 activity was markedly increased in the infarcted region acutely and persisted for at least 3 weeks post reperfusion.52,53 Therefore, targeted imaging with 111In-RP748 has demonstrated integrin αvβ3 activation early post infarction, suggesting a role for this technique in early detection of angiogenesis as well as for detection of chronic ischemia (Fig. 43-5).53
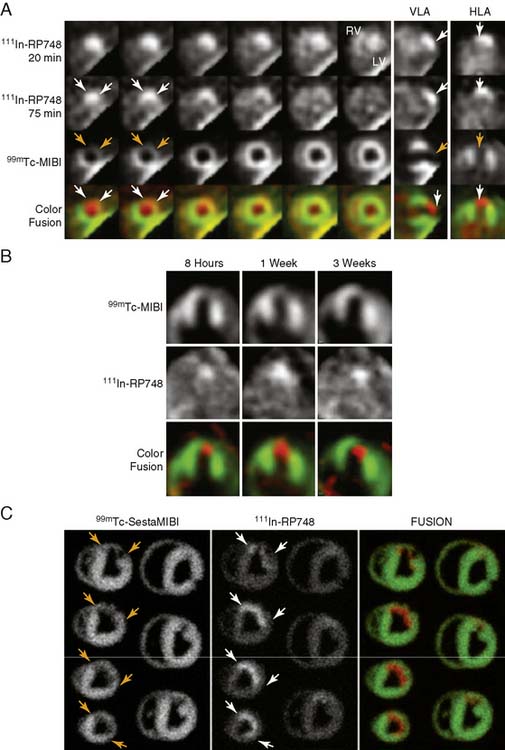
Figure 43-5 In vivo and ex vivo 111In-RP748 and 99mTc-sestamibi (99mTc-MIBI) images from dogs with chronic infarction. (A) Serial in vivo 111In-RP748 SPECT short-axis, vertical long-axis (VLA), and horizontal long-axis (HLA) images in a dog 3 weeks after LAD infarction at 20 minutes and 75 minutes after injection in standard format (Fig. 43-5A). 111In-RP748 SPECT, images were registered with 99mTc-MIBI perfusion images (third row). The 75-minute 111In-RP748 SPECT images were colored red and fused with MIBI images (green) to better demonstrate localization of 111In-RP748 activity within the heart (color fusion, bottom row). Right ventricular (RV) and left ventricular (LV) blood pool activity are seen at 20 minutes. White arrows indicate region of increased 111In-RP748 uptake in anterior wall. This corresponds to the anteroapical 99mTc-MIBI perfusion defect (orange arrow). (B) Sequential 99mTc-MIBI (top row) and 111In-RP748 in vivo SPECT HLA images at 90 minutes after injection (middle row) from a dog at 8 hours (Acute), and 1 and 3 weeks after LAD infarction (Fig. 43-5B). Increased myocardial 111In-RP748 uptake is seen in anteroapical wall at all three time points, although appears to be maximal at 1 week post infarction. Color fusion 99mTc-MIBI (green) and 111In-RP748 (red) images (bottom row) demonstrate 111In-RP748 uptake within 99mTc-MIBI perfusion defect. Ex vivo 99mTc-sestamibi (left) and 111In-RP748 (center) images of myocardial slices from a dog 3 weeks after LAD occlusion, with color fusion image on right (Fig. 43-5C). (C) Short-axis slices are oriented with anterior wall on top, RV on left. Orange arrows indicate anterior location of nontransmural perfusion defect region, and white arrows indicate corresponding area of increased 111In-RP748 uptake.
(From Meoli DF, Sadeghi MM, Krassilnikova, S et al: Noninvasive imaging of myocardial angiogenesis following experimental myocardial infarction, J Clin Invest 113:1684–1691, 2004. Reprinted with permission.)
Other experiments, utilizing a technetium-99m (99mTc)-labeled peptide, NC100692, in the rodent model of hindlimb ischemia for targeting of αvβ3 integrin have also been carried out and support the value of integrin imaging in models of peripheral arterial disease.54 The recent imaging of αvβ3 integrin by the PET imaging tracer 18F-galakto-RGD in a 35-year-old patient with a transmural MI 2 weeks prior demonstrates the feasibility of detecting angiogenesis in the myocardium in humans (Fig. 43-6).55
Recently, a new biodegradable positron-emitting nanoprobe targeted at αvβ3 integrin has been designed for noninvasive imaging of angiogenesis with a PET-based system (Fig. 43-7).56 The nanoprobe has a core-shell architecture that allows radiolabeling with radiohalogens that are linked to the core to protect them from dehalogenation. The terminal ends of the outer shell are covalently linked to cyclic-RGD peptides to confer specificity to αvβ3 integrin. This nanoprobe revealed enhanced binding of αvβ3 integrin using in vitro and cellular assays. The nanoprobe demonstrated favorable biodistribution when compared with untargeted probe in rodents, with a slight increase in uptake in phagocytic-rich organs like the spleen, liver, and kidneys. Using a bromine-76 (76Br)-labeled derivative of the nanoprobe, the investigators targeted αvβ3 integrin in a murine model of hindlimb ischemia. In vivo PET imaging revealed specificity of the probe to the ischemic limb, using the nonischemic limb and a version of the probe lacking the cyclic RGD peptides for controls. Ex vivo imaging and histologic analysis allowed for quantification of the radioactivity, leading to further association of the probe to areas of increased αvβ3 integrin expression and new vessel formation. The application of nanotechnology to angiogenesis imaging is an exciting new area of research, but further studies will be required to assess the feasibility of applying this system to the in vivo imaging of angiogenesis in the myocardium.
VENTRICULAR REMODELING
Ventricular remodeling is a complex biological process that involves inflammation, angiogenesis, repair, and healing, with specific biochemical and structural alterations in the myocardial infarct and periinfarct regions as well as remote regions (Fig. 43-8).57,58 The process is one of adaptation to form a scar that allows a degree of mechanical stability. The remodeling process involves several key cell types and structural elements, including myocardial cells, endothelial cells, inflammatory cells, and the extracellular matrix. Early in the first weeks after an MI, an innate immune response initiates a complex process of wound healing in the necrotic tissue. This process evolves into a more chronic remodeling process that can involve hypertrophy, chamber dilation, and (depending on the success of healing or lack thereof) heart failure.
Matrix metalloproteinases (MMPs) are a family of zinc-containing enzymes that play a key role in ventricular remodeling by degradation of the extracellular matrix.59,60 There are over 25 types of MMPs, and each can be distributed into subgroups based on substrate specificity and molecular structure.61 Despite apparent differences between various MMPs, there is often substrate overlap among the subgroups. Moreover, the zinc-binding motif sequence homology provides fairly conserved structure to the catalytic regions of all the family members. Because of the overlap’s substrate specificity, regulation of the various MMPs is critical to preventing pathologic phenomena. As such, MMPs are tightly regulated on several levels via transcriptional, posttranscriptional, and posttranslational mechanisms (Fig. 43-9). These regulatory mechanisms may serve as potential targets for disrupting MMP expression or activity. With regard to ventricular remodeling, MMPs appear to play an integral role in infarct expansion and left ventricular dilation. Gene deletion of MMPs has been demonstrated to have some cardioprotective effects from ventricular dilation and rupture post-infarct.62 Pharmacologic inhibition of MMPs has also been shown to decrease left ventricular dilation in infarct models.63–65
Another enzyme, factor XIII, has been shown to be crucial in organizing the new matrix of the scar by involvement with extracellular matrix turnover and regulation of inflammatory cascades.66,67 Mice with decreased levels of factor XIII demonstrate increased ventricular dilation and postinfarct rupture. Patients with infarct rupture were demonstrated to have lower levels of factor XIII in their myocardium. Factor XIII is activated by thrombin and often decreased in the setting of acute MI in part because of therapeutic inhibition of thrombin. It has been hypothesized that supplementing factor XIII activity may have a beneficial role in postinfarct remodeling.
A critical system that is locally activated during ventricular remodeling and contributes to the progression to heart failure is the renin-angiotensin system (Fig. 43-10).68,69 As healing and remodeling are unsuccessful in the failing heart, there is increased expression of prorenin, renin, and angiotensin-converting enzyme (ACE). Activation of this system through signaling pathways mediated by the angiotensin II type I receptor (AT1) leads to myocyte hypertrophy, interstitial and perivascular collagen deposition, and myocyte apoptosis.68 Inhibition of this pathway has been demonstrated to reverse the functional abnormalities associated with this negative remodeling.
RADIOTRACER-BASED IMAGING OF LEFT VENTRICULAR REMODELING
MMPs
By radiolabeling molecules that target MMPs, like pharmacologic inhibitors that specifically bind to the catalytic domain, MMP activation post infarct can be visualized in vivo (Fig. 43-11).70 Initial studies involved nonimaging techniques with 111In-labeled broad-spectrum MMP inhibitor (RP782), a molecule that selectively targets activated MMPs. This MMP-targeted agent demonstrated a favorable biodistribution in a murine model of MI. One week after MI, 111In-RP782 localized primarily within the infarct region, although a lesser increase in retention was seen in the remote noninfarcted regions of the heart, consistent with global MMP activation and remodeling.
Factor XIII
111In-DOTA-FXIII is a radiolabeled glutaminase factor XIII substrate analog that factor XIII cross-links to extracellular matrix proteins (Fig. 43-12).67 111In-DOTA-FXIII has been shown to accumulate in areas of increased factor XIII activity, using microSPECT/CT imaging in a murine model of MI. Mice treated with factor XIII intravenously exhibited increased factor XIII activity as reflected by 111In-DOTA-FXIII in the infarct zone. The mice also demonstrated more rapid inflammatory turnover of neutrophils and increased recruitment of macrophages to the site of infarction. There was also increased collagen synthesis and capillary density in the factor XIII–treated animals, suggesting improved healing after infarction. The 111In-labeled peptide substrate was demonstrated to be decreased in myocardial infarcts of animals treated with the direct thrombin inhibitor dalteparin. Moreover, dalteparin treatment increased the risk of infarct rupture. These experimental studies suggest there may be a role to image for factor XIII activity in an individual with MI and to therapeutically supplement activity where indicated to assist with positive ventricular remodeling, although further study is needed.
ACE Inhibitors and AT1 Antagonists
A number of ACE inhibitors and AT1 antagonists have been radiolabeled for molecular imaging techniques.69 In a study of explanted hearts from patients with ischemic cardiomyopathy, 18F-fluorobenzoyl-lisinopril was used to assess ACE levels in infracted myocardium and fibrosed tissue (Fig. 43-13).71 The study demonstrated that the radiolabeled ACE inhibitor bound with some degree of specificity to areas adjacent to the infarct. Other studies using AT1 antagonists have demonstrated a differential between ACE activity and AT1 levels.72 In an ovine model of heart failure, ACE activity was primarily in the vascular endothelium while AT1 was up-regulated in the myofibroblasts of the infarct region. In a murine model of acute MI, a 99mTc-labeled AT1 receptor peptide analog was developed and demonstrated specificity to the myofibroblasts that localized to the infarct region in the weeks following the infarction. These early studies suggest the changes in the renin-angiotensin system that take place within an infarction may be utilized to identify those at risk for developing significant heart failure after MI. Much more work is needed to assess the feasibility of these agents for imaging of post-infarction remodeling in clinical trials.
Integrin αvβ3
Because collagen deposition and fibrosis in the failing heart appear to be mediated by myofibroblasts, markers that indicate increased myofibroblast recruitment and activity are of interest to the field.73 Myofibroblasts demonstrate an up-regulation of angiotensin receptors as mentioned above; however, they demonstrate an up-regulation of integrin moieties as well.72,74 Taking advantage of this molecular event, a recent study used the 99mTc-labeled cy5.5-RGD peptide analog, CRIP, to image up-regulated αvβ3 integrins in a murine model of MI (Fig. 43-14).75 Fluorescence imaging and histologic analysis of explanted hearts revealed that CRIP co-localized to areas of myofibroblasts in the infarct region. Utilizing CT for registration, in vivo microSPECT analysis confirmed localization of the CRIP to the infarct and border zones. Maximum signal intensity was in the first 2 weeks post-infarction and then tapered at 4 and 12 weeks. A scrambled CRIP analog did not show uptake in the region, supporting the specificity of the CRIP for αvβ3 integrin binding. Moreover, a subgroup of animals were treated with either captopril or a combination of captopril and losartan in an attempt to positively affect ventricular remodeling and visualize the effect on the CRIP–αvβ3 integrin signal. Echocardiography supported smaller infarct size and improved ejection fraction in the treated animals. Ex vivo SPECT imaging of CRIP revealed lower signal intensity images in the treated hearts. This preliminary study suggests not only that critical changes in the ventricular myocardium after MI can be imaged using a molecular approach, but that a response to remodeling therapy may also be assessed.
INFLAMMATION
Initial attempts to image inflammatory processes involved radiolabeling leukocytes with 99mTc or 111In. These techniques require removal of blood and in vitro labeling prior to reinjection into the blood stream for imaging.76,77 The concern over this in vitro labeling approach is the labeling often results in nonspecific activation of the cells, which in turn may interfere with cellular localization in vivo. Gallium-67-labeled, citrate has also been used but carries the same concerns regarding nonspecificity.78 18F-FDG PET imaging takes advantage of increased metabolic activity of inflammatory cells, but changes in glucose uptake can be associated with other tissues and disease processes including tumors.79,80 Thus, there is a tremendous amount of interest in developing more-specific noninvasive imaging techniques to detect inflammation in myocardium.
RADIOTRACER-BASED IMAGING OF INFLAMMATORY-MEDIATED PROCESSES
Antimyosin Antibodies
Injury to myocytes in the setting of inflammation leads to the disruption of cellular membranes and the release of myosin heavy chain. In order to take advantage of this extracellular exposure of myosin in the setting of inflammation and necrosis, monoclonal antibody to myosin was generated in hopes of applying this for imaging. Early attempts to visualize myosin utilized 111In-labeled antimyosin antibodies to visualize myocyte damage in MI.81 Other studies utilized 99mTc-labeled monoclonal antibody fragments to quantitate the degree of myosin exposure in patients in the setting of acute MI and correlate it with necrosis.82 Inflammation associated with myocarditis, an inflammatory process not associated with ischemia, was also carried out using 111In-anti-myosin antibodies.83,84 Utilizing anti-myosin antibody imaging, patients with dilated cardiomyopathy and lower ejection fractions revealed positive studies, suggesting a role for this for stratifying appropriate patients for cardiac transplantation. Though these initial studies showed promise, the background antibody binding to necrotic debris in the cell was high, and therefore the specificity of 111In-labeled anti-myosin antibody turned out to be very low (25% to 50%).85
Antitenascin-C Antibody
A monoclonal antibody against the extracellular matrix protein, tenascin-C, which appears to be involved in wound healing and inflammation, has been identified as another potential imaging agent for detection of inflammation. In rodent models of myocarditis, 111In-labeled anti-tenascin-C localizes to the sites of myocardial inflammation.86 Using a dual-isotope SPECT approach with 111In-anti-tenascin-C and 99mTc-sestamibi, the antibody is localized to the injured septal wall by in vivo imaging. More recently, 111In-antitenascin-C monoclonal antibody fragments have been used in a dual-isotope SPECT imaging approach with 99mTc-MIBI to study in vivo expression of tenascin-C in a rodent model of MI (Fig. 43-15).87 In this study, 111In-anti-tenascin-C activity was associated with the 99mTc-MIBI perfusion defect, although not in sham animals, supporting this agent as a possible marker for inflammation associated with MI. Though much work is needed, the authors postulate that quantifying tenascin binding using a similar approach might allow for the evaluation of ventricular repair.
LTB4 Receptor
LTB4 is a lipid mediator synthesized from arachidonic acid and secreted by neutrophils, macrophages, and endothelial cells as a potent chemotactic agent.88,89 The LTB4 receptor can be found on neutrophils, and signaling through this receptor stimulates endothelial adhesion and superoxide production. Recently a radiolabeled LTB4 receptor antagonist, 99mTc-RP517, was developed for in vivo imaging of inflammation.90,91 99mTc-RP517 localized to sites of inflammation induced by Staphylococcus aureus and Escherichia coli infection and chemical (phorbol ester)-induced bowel inflammation.
When prepared with human peripheral whole blood in vitro, fluorinated RP517 localized to neutrophils by fluorescence-activated cell sorter (FACs) analysis.92 This confirmed the potential to specifically label human blood neutrophils with 99mTc-labeled RP517. In an attempt to characterize the in vivo imaging ability of 99mTc-RP517, a canine model of postischemic myocardial inflammation was utilized. 99mTc-RP517 was injected into open-chest dogs before occlusion and reperfusion. There was an inverse relationship between radiotracer uptake and occlusion flow, suggesting localization of the imaging agent to the site of ischemic inflammation (Fig. 43-16). Ex vivo segment analysis revealed that 99mTc-RP517 correlated with the neutrophil enzyme myeloperoxidase. Intramyocardial injection of tumor necrosis factor α (TNF-α) also correlated with 99mTc-RP517 uptake and concomitant myeloperoxidase activity, again supporting localization to the site of inflammation. One concern regarding the application of 99mTc-RP517 is the lipophilic nature of the molecule, resulting in high hepatobiliary clearance and thus large amounts of gastrointestinal uptake. To overcome this, alternative constructs of the LTB4 antagonist are currently being evaluated.93
CELL DEATH
Apoptosis Versus Necrosis
Apoptosis is the physiologic process of programmed cell death whereby organisms selectively target cells to be eliminated when they are no longer needed. The cardiovascular pathologies of cardiomyopathy, heart failure, myocarditis, and MI are associated with increased levels of apoptosis, particularly in the myocyte. There is a subset of cell death that occurs as an outcome of these pathologic processes considered to be outside of programmed cellular mechanisms, termed necrosis. A recent study evaluating a role of apoptosis and necrosis in the setting of acute MI revealed a potential therapeutic role for cyclosporine.94 The intervention is hypothesized to minimize periinfarct, reperfusion-related cell death that takes place in the setting of revascularization. It is estimated that 30% of cardiomyocytes in the injured myocardium become apoptotic as a result of ischemia, reperfusion injury, and animal models of acute infarction demonstrate that up to 50% of the final size of the infarct can be related to lethal reperfusion injury.95,96 Other animal studies demonstrate that inhibition of apoptosis with caspase-inhibitors is cardioprotective.97–99 There are also data that suggest early apoptosis may be the pathologic substrate leading from ischemia to necrosis.100 An ability to assess cell death anywhere along the spectrum of apoptosis to necrosis would allow investigators to fine-tune a therapeutic regimen and optimize clinical outcome. In targeting these pathologies for new interventions, it is apparent that better in vivo imaging techniques for detection of apoptosis are required.
The earliest studies of apoptosis evolved around histologic assessment of the cells undergoing cell death. These descriptions included the microscopic visualization of chromatin condensation, dissolution of nuclear membrane, nuclear shrinkage, and formation of apoptotic bodies that were cleared by phagocytic cells.101–103 Over time, it became evident that programmed cell death is central to the development and maintenance of homeostasis for any multicellular organism (Fig. 43-17).104,105 In addition, cell death appears to play a role in the pathology of various disease states.106 Depending on the initiating signals, there are two major pathways for cell death: intrinsic and extrinsic.105 The intrinsic pathway is generated from within the cell through DNA damage, mitochondrial signals, and oncogene activation, leading to activation of caspase enzymes, cysteine proteases that cleave after aspartate residues. The extrinsic pathway is initiated through extracellular signals that target cell membrane receptors like Fas, a death receptor. The culmination of this event through either pathway is the activation of a key effector, caspase-3.107
Soon after the activation of caspase-3, the energy-dependent asymmetric distribution of phospholipids that enables the definition of various subregions within the lipid bilayer of cell membranes is lost. This leads to increased phosphatidyl serine (PS) on the outer cell membrane from its typical location on the inner cell membrane.108 In part, this is the result of increased calcium levels and decreased amounts of ATP that block the translocase enzyme responsible for maintenance of PS. The exposure of PS on the surface of the cell makes it a target for binding the protein annexin V.109 Annexin V binds to PS in a calcium-dependent manner. This has lead to an in vitro assay of Fas-ligand-initiated cell death through binding of annexin V.108,110
The first application of annexin binding to identify phosphatidyl serine on the surface of cells in a cardiovascular model came from a mouse model of acute MI.111 In this study, the left anterior descending coronary artery of a series of mice was ligated shortly after the injection of biotinylated annexin V. Immunohistochemical analysis of the tissue distal to the site of ligation revealed annexin A5 binding in an area of cell death. DNA laddering confirmed programmed cell death to be occurring in the same region as the annexin A5 binding.
As myocardial ischemia or infarction persists, cells move from early apoptotic signals to complete necrosis. Breakdown of mitochondrial respiration and loss of membrane potential lead to the accumulation of calcium in the mitochondria of infarcted or severely injured myocardium.112,113 With loss of membrane potential, cellular structures also begin to dissipate. Positively charged histones and other organelle proteins are exposed from the protection of their membrane barriers. These changes in early necrotic tissue have been utilized for imaging techniques that seek to identify early necrosis in acute MIs and are discussed in more detail below.
RADIOTRACER-BASED IMAGING IN APOPTOSIS AND NECROSIS
Annexin V
Initial studies utilized 99mTc-labeled annexin A5 for imaging the distribution of cells expressing PS noninvasively with a standard gamma camera. Radiolabeling involved derivatization of annexin A5 with hydrazinonicotinamine (HYNIC), which binds to reduced 99mTc.114 The initial studies were carried out in mice with fulminant hepatic apoptosis through the injection of an anti-Fas antibody, which initiates an apoptotic cascade, particularly in hepatocytes.115 Concomitant TUNEL studies confirmed localization of annexin A5 with apoptotic cells.
99mTc-labeled annexin V was subsequently utilized in humans to detect in vivo cell death in patients presenting with MI (Fig. 43-18).116 Patients presenting with their first MI within 6 hours of symptom onset underwent standard revascularization with percutaneous intervention. Within 2 hours of revascularization, SPECT imaging was performed utilizing 99mTc-MIBI annexin V. This was followed by perfusion imaging 6 to 8 weeks after discharge using 99mTc-sestamibi. Regional retention of 99mTc-labeled annexin V correlated with the perfusion defect identified 6 to 8 weeks after discharge, providing a proof of concept that annexin V imaging can be utilized for noninvasive detection of myocardial cell death.
Heart transplant rejection is characterized by perivascular and interstitial mononuclear inflammatory infiltrates associated with myocyte apoptosis and necrosis.117 In a study of 18 patients undergoing apoptotic imaging within 1 year of cardiac transplantation, annexin V retention correlated with the severity of rejection.118 Patients with a negative scan had a concomitant negative biopsy. Of the five patients with a positive scan, three patients demonstrated regional uptake while two patients demonstrated diffuse uptake. The annexin V scans correlated with the degree of severity of rejection by biopsy specimens. The authors suggested that serial annexin V imaging for apoptotic cells could be used as a surrogate for detection of allograft rejection in place of serial biopsies in patients following heart transplantation.
Myocarditis is another pathologic condition where apoptosis is known to occur.119 In a rat model of autoimmune myocarditis, 99mTc-labeled annexin V retention corresponded to histologic TUNEL staining for areas of myocardial apoptosis. Interestingly, 99mTc-labeled annexin V–positive areas could be differentiated from areas of inflammation identified by carbon-14 (14C)-labeled deoxyglucose, reflecting foci of inflammation. This suggests that one could potentially differentiate between inflammation and active apoptosis with dual-isotope molecular imaging. To date, no studies have attempted to employ this technique in conjunction with FDG PET in human cases of myocarditis.
Caspase Inhibitors
Because phosphatidyl serine can be exposed on the surface of cells in physiologic conditions other than apoptosis, there is interest in developing more-specific apoptosis tracers. Recently, caspase-3 inhibitors have been synthesized and labeled with 18F as potential PET tracers for in vivo imaging of apoptosis (see Fig. 43-17).120,121 These caspase-3-targeted tracers have shown favorable biodistribution and clearance. MicroPET imaging in a murine model of hepatic apoptosis has shown specificity of the tracer to the liver; however, more studies are needed to assess binding relative to activated caspase density. In addition, further analysis in cardiovascular models will be necessary to determine feasibility of utilizing this new class of tracers for cardiac applications in humans.
Pyrophosphate and Glucarate
The in vivo noninvasive detection MI will allow for early diagnosis and treatment in patients when electrocardiographic changes may not be evident, or when biomarkers may not distinguish between ischemic injury associated with acute plaque rupture that may be treatable with mechanical revascularization versus unstable angina and demand-related ischemia. In addition to visualizing apoptosis, several studies have demonstrated that certain agents allow for the visualization of ongoing myocardial necrosis as a mechanism of identifying acute infarction, potentially even in the presence of prior MI. 99mTc-labeled pyrophosphate has been shown to bind to areas of necrosis and is thought to bind exposed mitochondrial calcium.112,113 99mTc-pyrophosphate has a moderate degree of sensitivity for acute infarction, depending on the presence of Q-wave infarction or a non-ST-elevation infarction.122 The specificity for acute MI is considered to be between 60% and 80%. The primary reason why 99mTc-pyrophosphate has not gained widespread clinical use is the limitation in the detection of early infarction. In fact, depending on the residual degree of perfusion to the infarct zone, the test may not be positive for the first 24 hours.
99mTc-glucarate imaging provides an alternative to 99mTc-pyrophosphate imaging for the detection of acute infarction.113 99mTc-glucarate enters the necrotic cells by passive diffusion following breakdown of the sarcolemma, then binds to exposed histones in the nucleus of the myocytes. Canine models for ischemia and infarction reveal a high affinity of 99mTc-glucarate for necrotic tissue over ischemic but viable myocardium.123 Moreover, establishing partial reperfusion did not inhibit the ability to detect acute infarct in canines (Fig. 43-19).124 In a rabbit model of infarction, 99mTc-glucarate did not accumulate in areas of ischemia and could be imaged in areas of infarction as early as 10 minutes after reperfusion and within 30 to 60 minutes in nonreperfused zones.125 Initial data in patients revealed that 99mTc-glucarate is able to noninvasively diagnose MI in patients presenting with chest pain, with a sensitivity that is dependent on the onset of symptoms, specifically within the first 9 hours of symptom onset.126 99mTc-glucarate also demonstrates rapid blood clearance and good target-to-background signal. 99mTc-glucarate imaging is currently under investigation as a tool to detect early infarction in a number of clinical trials.
1. Pichler A., Piwnica-Worms D. Overview of cardiovascular molecular imaging. In: Gropler R.J., Glover D.K., Sinusas A.J., et al, editors. Cardiovascular molecular imaging. New York: Informa Healthcare U.S.A., Inc; 2007:1-8.
2. Sinusas A.J., Bengel F., Nahrendorf M., et al. Multimodality cardiovascular molecular imaging, Part I. Circ Cardiovasc Imaging. 2008;1:244-256.
3. Hansson G.K. Inflammation, atherosclerosis, and coronary artery disease. N Engl J Med. 2005;352:1685-1695.
4. Weber C., Zernecke A., Libby P. The multifaceted contributions of leukocyte subsets to atherosclerosis: lessons from mouse models. Nat Rev Immunol. 2008;8:802-815.
5. Fam N.P., Verma S., Kutryk M., et al. Clinician guide to angiogenesis. Circulation. 2003;108:2613-2618.
6. Cai W., Chen X. Multimodality molecular imaging of tumor angiogenesis. J Nucl Med. 2008;49(Suppl 2):113S-128S.
7. Kerbel R.S. Tumor angiogenesis. N Engl J Med. 2008;358:2039-2049.
8. Sasayama S., Fujita M. Recent insights into coronary collateral circulation. Circulation. 1992;85:1197-1204.
9. Dufraine J., Funahashi Y., Kitajewski J. Notch signaling regulates tumor angiogenesis by diverse mechanisms. Oncogene. 2008;27:5132-5137.
10. Shweiki D., Itin A., Soffer D., et al. Vascular endothelial growth factor induced by hypoxia may mediate hypoxia-initiated angiogenesis. Nature. 1992;359:843-845.
11. Lee S.H., Wolf P.L., Escudero R., et al. Early expression of angiogenesis factors in acute myocardial ischemia and infarction. N Engl J Med. 2000;342:626-633.
12. Semenza G.L. Life with oxygen. Science. 2007;318:62-64.
13. Brogi E., Schatteman G., Wu T., et al. Hypoxia-induced paracrine regulation of vascular endothelial growth factor receptor expression. J Clin Invest. 1996;97:469-476.
14. Banai S., Jaklitsch M.T., Shou M., et al. Angiogenic-induced enhancement of collateral blood flow to ischemic myocardium by vascular endothelial growth factor in dogs. Circulation. 1994;89:2183-2189.
15. Li J., Brown L.F., Hibberd M.G., et al. VEGF, flk-1, and flt-1 expression in a rat myocardial infarction model of angiogenesis. Am J Physiol. 1996;270:H1803-H1811.
16. Hu C.J., Iyer S., Sataur A., et al. Differential regulation of the transcriptional activities of hypoxia-inducible factor 1 alpha (HIF-1alpha) and HIF-2alpha in stem cells. Mol Cell Biol. 2006;26:3514-3526.
17. Ferrara N., Gerber H.P., LeCouter J. The biology of VEGF and its receptors. Nat Med. 2003;9:669-676.
18. Giordano F.J., Ping P., McKirnan M.D., et al. Intracoronary gene transfer of fibroblast growth factor-5 increases blood flow and contractile function in an ischemic region of the heart. Nat Med. 1996;2:534-539.
19. Simons M., Annex B.H., Laham R.J., et al. Pharmacological treatment of coronary artery disease with recombinant fibroblast growth factor-2: double-blind, randomized, controlled clinical trial. Circulation. 2002;105:788-793.
20. Grines C.L., Watkins M.W., Helmer G., et al. Angiogenic Gene Therapy (AGENT) trial in patients with stable angina pectoris. Circulation. 2002;105:1291-1297.
21. Henry T.D., Annex B.H., McKendall G.R., et al. The VIVA trial: Vascular endothelial growth factor in Ischemia for Vascular Angiogenesis. Circulation. 2003;107:1359-1365.
22. Stewart D.J., Hilton J.D., Arnold J.M., et al. Angiogenic gene therapy in patients with nonrevascularizable ischemic heart disease: a phase 2 randomized, controlled trial of AdVEGF(121) (AdVEGF121) versus maximum medical treatment. Gene Ther. 2006;13:1503-1511.
23. Kastrup J., Jorgensen E., Ruck A., et al. Direct intramyocardial plasmid vascular endothelial growth factor-A165 gene therapy in patients with stable severe angina pectoris A randomized double-blind placebo-controlled study: the Euroinject One trial. J Am Coll Cardiol. 2005;45:982-988.
24. Hanahan D. Signaling vascular morphogenesis and maintenance. Science. 1997;277:48-50.
25. Oliner J., Min H., Leal J., et al. Suppression of angiogenesis and tumor growth by selective inhibition of angiopoietin-2. Cancer Cell. 2004;6:507-516.
26. Zacchigna S., Tasciotti E., Kusmic C., et al. In vivo imaging shows abnormal function of vascular endothelial growth factor-induced vasculature. Hum Gene Ther. 2007;18:515-524.
27. Gale N.W., Dominguez M.G., Noguera I., et al. Haploinsufficiency of delta-like 4 ligand results in embryonic lethality due to major defects in arterial and vascular development. Proc Natl Acad Sci U S A. 2004;101:15949-15954.
28. Lobov I.B., Renard R.A., Papadopoulos N., et al. Delta-like ligand 4 (Dll4) is induced by VEGF as a negative regulator of angiogenic sprouting. Proc Natl Acad Sci U S A. 2007;104:3219-3224.
29. Noguera-Troise I., Daly C., Papadopoulos N.J., et al. Blockade of Dll4 inhibits tumour growth by promoting non-productive angiogenesis. Nature. 2006;444:1032-1037.
30. Sainson R.C., Harris A.L. Anti-Dll4 therapy: can we block tumour growth by increasing angiogenesis? Trends Mol Med. 2007;13:389-395.
31. Xiong J.P., Stehle T., Diefenbach B., et al. Crystal structure of the extracellular segment of integrin alphavbeta3. Science. 2001;294:339-345.
32. Brooks P.C., Montgomery A.M., Rosenfeld M., et al. Integrin alpha v beta 3 antagonists promote tumor regression by inducing apoptosis of angiogenic blood vessels. Cell. 1994;79:1157-1164.
33. Schwartz M.A., Schaller M.D., Ginsberg M.H. Integrins: emerging paradigms of signal transduction. Annu Rev Cell Dev Biol. 1995;11:549-599.
34. Hynes R.O. Integrins: bidirectional, allosteric signaling machines. Cell. 2002;110:673-687.
35. Clyman R.I., Mauray F., Kramer R.H. Beta 1 and beta 3 integrins have different roles in the adhesion and migration of vascular smooth muscle cells on extracellular matrix. Exp Cell Res. 1992;200:272-284.
36. Li J., Brown L.F., Laham R.J., et al. Macrophage-dependent regulation of syndecan gene expression. Circ Res. 1997;81:785-796.
37. Bhagwat S.V., Lahdenranta J., Giordano R., et al. CD13/APN is activated by angiogenic signals and is essential for capillary tube formation. Blood. 2001;97:652-659.
38. Bergers G., Song S., Meyer-Morse N., et al. Benefits of targeting both pericytes and endothelial cells in the tumor vasculature with kinase inhibitors. J Clin Invest. 2003;111:1287-1295.
39. Lake Tahoe invitation meeting 2002. J Nucl Cardiol. 2003;10:223-257.
40. Lu E., Wagner W.R., Schellenberger U., et al. Targeted in vivo labeling of receptors for vascular endothelial growth factor: approach to identification of ischemic tissue. Circulation. 2003;108:97-103.
41. Rodriguez-Porcel M., Cai W., Gheysens O., et al. Imaging of VEGF receptor in a rat myocardial infarction model using PET. J Nucl Med. 2008;49:667-673.
42. Willmann J.K., Chen K., Wang H., et al. Monitoring of the biological response to murine hindlimb ischemia with 64Cu-labeled vascular endothelial growth factor-121 positron emission tomography. Circulation. 2008;117:915-922.
43. Wu J.C., Inubushi M., Sundaresan G., et al. Positron emission tomography imaging of cardiac reporter gene expression in living rats. Circulation. 2002;106:180-183.
44. Inubushi M., Wu J.C., Gambhir S.S., et al. Positron-emission tomography reporter gene expression imaging in rat myocardium. Circulation. 2003;107:326-332.
45. Bengel F.M., Anton M., Richter T., et al. Noninvasive imaging of transgene expression by use of positron emission tomography in a pig model of myocardial gene transfer. Circulation. 2003;108:2127-2133.
46. Wu J.C., Chen I.Y., Wang Y., et al. Molecular imaging of the kinetics of vascular endothelial growth factor gene expression in ischemic myocardium. Circulation. 2004;110:685-691.
47. Sipkins D.A., Cheresh D.A., Kazemi M.R., et al. Detection of tumor angiogenesis in vivo by alphaVbeta3-targeted magnetic resonance imaging. Nat Med. 1998;4:623-626.
48. Haubner R., Wester H.J., Weber W.A., et al. Noninvasive imaging of alpha(v)beta(3) integrin expression using 18F-labeled RGD-containing glycopeptide and positron emission tomography. Cancer Res. 2001;61:1781-1785.
49. Haubner R., Wester H.J., Burkhart F., et al. Glycosylated RGD-containing peptides: tracer for tumor targeting and angiogenesis imaging with improved biokinetics. J Nucl Med. 2001;42:326-336.
50. Harris T.D., Kalogeropoulos S., Nguyen T., et al. Design, synthesis, and evaluation of radiolabeled integrin alpha v beta 3 receptor antagonists for tumor imaging and radiotherapy. Cancer Biother Radiopharm. 2003;18:627-641.
51. Sadeghi M., Krassilnikova S., Zhang J., et al. Imaging of avb3 integrin in vascular injury: Does this reflect increased integrin expression or activation? Circulation. 2008;108:404.
52. Meoli D.F., Sadeghi M.M., Krassilnikova S., et al. Noninvasive imaging of myocardial angiogenesis following experimental myocardial infarction. J Clin Invest. 2004;113:1684-1691.
53. Kalinowski L., Dobrucki L.W., Meoli D.F., et al. Targeted imaging of hypoxia-induced integrin activation in myocardium early after infarction. J Appl Physiol. 2008;104:1504-1512.
54. Su H., Hu X., Bourke B., et al. Detection of myocardial angiogenesis in chronic infarction with a novel technetium-99m labeled peptide targeted at avb3 integrin. Circulation. 2003;108:278-279.
55. Makowski M.R., Ebersberger U., Nekolla S., et al. In vivo molecular imaging of angiogenesis, targeting alphavbeta3 integrin expression, in a patient after acute myocardial infarction. Eur Heart J. 2008;29:2201.
56. Almutairi A., Rossin R., Shokeen M., et al. Biodegradable dendritic positron-emitting nanoprobes for the noninvasive imaging of angiogenesis. Proc Natl Acad Sci U S A. 2009;106:685-690.
57. Weber K.T. Extracellular matrix remodeling in heart failure: a role for de novo angiotensin II generation. Circulation. 1997;96:4065-4082.
58. Sutton M.G., Sharpe N. Left ventricular remodeling after myocardial infarction: pathophysiology and therapy. Circulation. 2000;101:2981-2988.
59. Creemers E.E., Cleutjens J.P., Smits J.F., et al. Matrix metalloproteinase inhibition after myocardial infarction: a new approach to prevent heart failure? Circ Res. 2001;89:201-210.
60. Spinale F.G. Matrix metalloproteinases: regulation and dysregulation in the failing heart. Circ Res. 2002;90:520-530.
61. Sternlicht M.D., Werb Z. How matrix metalloproteinases regulate cell behavior. Annu Rev Cell Dev Biol. 2001;17:463-516.
62. Ducharme A., Frantz S., Aikawa M., et al. Targeted deletion of matrix metalloproteinase-9 attenuates left ventricular enlargement and collagen accumulation after experimental myocardial infarction. J Clin Invest. 2000;106:55-62.
63. Rohde L.E., Ducharme A., Arroyo L.H., et al. Matrix metalloproteinase inhibition attenuates early left ventricular enlargement after experimental myocardial infarction in mice. Circulation. 1999;99:3063-3070.
64. Lindsey M.L., Gannon J., Aikawa M., et al. Selective matrix metalloproteinase inhibition reduces left ventricular remodeling but does not inhibit angiogenesis after myocardial infarction. Circulation. 2002;105:753-758.
65. Yarbrough W.M., Mukherjee R., Escobar G.P., et al. Selective targeting and timing of matrix metalloproteinase inhibition in post-myocardial infarction remodeling. Circulation. 2003;108:1753-1759.
66. Nahrendorf M., Hu K., Frantz S., et al. Factor XIII deficiency causes cardiac rupture, impairs wound healing, and aggravates cardiac remodeling in mice with myocardial infarction. Circulation. 2006;113:1196-1202.
67. Nahrendorf M., Aikawa E., Figueiredo J.L., et al. Transglutaminase activity in acute infarcts predicts healing outcome and left ventricular remodelling: implications for FXIII therapy and antithrombin use in myocardial infarction. Eur Heart J. 2008;29:445-454.
68. Aras O., Messina S.A., Shirani J., et al. The role and regulation of cardiac angiotensin-converting enzyme for noninvasive molecular imaging in heart failure. Curr Cardiol Rep. 2007;9:150-158.
69. Shirani J., Dilsizian V. Imaging left ventricular remodeling: targeting the neurohumoral axis. Nat Clin Pract Cardiovasc Med. 2008;2(Suppl 5):S57-S62.
70. Su H., Spinale F.G., Dobrucki L.W., et al. Noninvasive targeted imaging of matrix metalloproteinase activation in a murine model of postinfarction remodeling. Circulation. 2005;112:3157-3167.
71. Dilsizian V., Eckelman W.C., Loredo M.L., et al. Evidence for tissue angiotensin-converting enzyme in explanted hearts of ischemic cardiomyopathy using targeted radiotracer technique. J Nucl Med. 2007;48:182-187.
72. Shirani J., Narula J., Eckelman W.C., et al. Early imaging in heart failure: exploring novel molecular targets. J Nucl Cardiol. 2007;14:100-110.
73. Cleutjens J.P., Blankesteijn W.M., Daemen M.J., et al. The infarcted myocardium: simply dead tissue, or a lively target for therapeutic interventions. Cardiovasc Res. 1999;44:232-241.
74. Asano Y., Ihn H., Yamane K., et al. Increased expression of integrin alpha(v)beta3 contributes to the establishment of autocrine TGF-beta signaling in scleroderma fibroblasts. J Immunol. 2005;175:7708-7718.
75. van den Borne S.W., Isobe S., Verjans J.W., et al. Molecular imaging of interstitial alterations in remodeling myocardium after myocardial infarction. J Am Coll Cardiol. 2008;52:2017-2028.
76. Peters A.M., Danpure H.J., Osman S., et al. Clinical experience with 99mTc-hexamethylpropylene-amineoxime for labelling leucocytes and imaging inflammation. Lancet. 1986;2:946-949.
77. Peters A.M., Saverymuttu S.H. The value of indium-labelled leucocytes in clinical practice. Blood Rev. 1987;1:65-76.
78. Lavender J.P., Lowe J., Barker J.R., et al. Gallium 67 citrate scanning in neoplastic and inflammatory lesions. Br J Radiol. 1971;44:361-366.
79. Mochizuki T., Tsukamoto E., Kuge Y., et al. FDG uptake and glucose transporter subtype expressions in experimental tumor and inflammation models. J Nucl Med. 2001;42:1551-1555.
80. Kubota R., Yamada S., Kubota K., et al. Intratumoral distribution of fluorine-18-fluorodeoxyglucose in vivo: high accumulation in macrophages and granulation tissues studied by microautoradiography. J Nucl Med. 1992;33:1972-1980.
81. Johnson L.L., Seldin D.W., Becker L.C., et al. Antimyosin imaging in acute transmural myocardial infarctions: results of a multicenter clinical trial. J Am Coll Cardiol. 1989;13:27-35.
82. Khaw B.A., Gold H.K., Yasuda T., et al. Scintigraphic quantification of myocardial necrosis in patients after intravenous injection of myosin-specific antibody. Circulation. 1986;74:501-508.
83. Yasuda T., Palacios I.F., Dec G.W., et al. Indium 111-monoclonal antimyosin antibody imaging in the diagnosis of acute myocarditis. Circulation. 1987;76:306-311.
84. Dec G.W., Palacios I., Yasuda T., et al. Antimyosin antibody cardiac imaging: its role in the diagnosis of myocarditis. J Am Coll Cardiol. 1990;16:97-104.
85. Narula J., Khaw B.A., Dec G.W., et al. Diagnostic accuracy of antimyosin scintigraphy in suspected myocarditis. J Nucl Cardiol. 1996;3:371-381.
86. Sato M., Toyozaki T., Odaka K., et al. Detection of experimental autoimmune myocarditis in rats by 111In monoclonal antibody specific for tenascin-C. Circulation. 2002;106:1397-1402.
87. Odaka K., Uehara T., Arano Y., et al. Noninvasive detection of cardiac repair after acute myocardial infarction in rats by 111In Fab fragment of monoclonal antibody specific for tenascin-C. Int Heart J. 2008;49:481-492.
88. Ford-Hutchinson A.W. Regulation of leukotriene biosynthesis. Cancer Metastasis Rev. 1994;13:257-267.
89. Yokomizo T., Izumi T., Shimizu T. Leukotriene B4: metabolism and signal transduction. Arch Biochem Biophys. 2001;385:231-241.
90. Serhan C.N., Prescott S.M. The scent of a phagocyte: Advances on leukotriene b(4) receptors. J Exp Med. 2000;192:F5-F8.
91. Brouwers A.H., Laverman P., Boerman O.C., et al. A 99Tcm-labelled leukotriene B4 receptor antagonist for scintigraphic detection of infection in rabbits. Nucl Med Commun. 2000;21:1043-1050.
92. Riou L.M., Ruiz M., Sullivan G.W., et al. Assessment of myocardial inflammation produced by experimental coronary occlusion and reperfusion with 99mTc-RP517, a new leukotriene B4 receptor antagonist that preferentially labels neutrophils in vivo. Circulation. 2002;106:592-598.
93. van Eerd J.E., Oyen W.J., Harris T.D., et al. A bivalent leukotriene B(4) antagonist for scintigraphic imaging of infectious foci. J Nucl Med. 2003;44:1087-1091.
94. Piot C., Croisille P., Staat P., et al. Effect of cyclosporine on reperfusion injury in acute myocardial infarction. N Engl J Med. 2008;359:473-481.
95. Fliss H., Gattinger D. Apoptosis in ischemic and reperfused rat myocardium. Circ Res. 1996;79:949-956.
96. Yellon D.M., Hausenloy D.J. Myocardial reperfusion injury. N Engl J Med. 2007;357:1121-1135.
97. Yaoita H., Ogawa K., Maehara K., et al. Attenuation of ischemia/reperfusion injury in rats by a caspase inhibitor. Circulation. 1998;97:276-281.
98. Dumont E.A., Reutelingsperger C.P., Smits J.F., et al. Real-time imaging of apoptotic cell-membrane changes at the single-cell level in the beating murine heart. Nat Med. 2001;7:1352-1355.
99. Hayakawa Y., Chandra M., Miao W., et al. Inhibition of cardiac myocyte apoptosis improves cardiac function and abolishes mortality in the peripartum cardiomyopathy of Galpha(q) transgenic mice. Circulation. 2003;108:3036-3041.
100. Thimister P.W., Hofstra L., Liem I.H., et al. In vivo detection of cell death in the area at risk in acute myocardial infarction. J Nucl Med. 2003;44:391-396.
101. Wyllie A.H. Glucocorticoid-induced thymocyte apoptosis is associated with endogenous endonuclease activation. Nature. 1980;284:555-556.
102. Kerr J.F., Wyllie A.H., Currie A.R. Apoptosis: a basic biological phenomenon with wide-ranging implications in tissue kinetics. Br J Cancer. 1972;26:239-257.
103. Wyllie A.H., Kerr J.F., Currie A.R. Cell death: the significance of apoptosis. Int Rev Cytol. 1980;68:251-306.
104. Danial N.N., Korsmeyer S.J. Cell death: critical control points. Cell. 2004;116:205-219.
105. Riedl S.J., Shi Y. Molecular mechanisms of caspase regulation during apoptosis. Nat Rev Mol Cell Biol. 2004;5:897-907.
106. Green D.R., Kroemer G. The pathophysiology of mitochondrial cell death. Science. 2004;305:626-629.
107. Tait J.F. Imaging of apoptosis. J Nucl Med. 2008;49:1573-1576.
108. Martin S.J., Reutelingsperger C.P., McGahon A.J., et al. Early redistribution of plasma membrane phosphatidylserine is a general feature of apoptosis regardless of the initiating stimulus: inhibition by overexpression of Bcl-2 and Abl. J Exp Med. 1995;182:1545-1556.
109. Koopman G., Reutelingsperger C.P., Kuijten G.A., et al. Annexin V for flow cytometric detection of phosphatidylserine expression on B cells undergoing apoptosis. Blood. 1994;84:1415-1420.
110. van Engeland M., Ramaekers F.C., Schutte B., et al. A novel assay to measure loss of plasma membrane asymmetry during apoptosis of adherent cells in culture. Cytometry. 1996;24:131-139.
111. Dumont E.A., Hofstra L., van Heerde W.L., et al. Cardiomyocyte death induced by myocardial ischemia and reperfusion: measurement with recombinant human annexin-V in a mouse model. Circulation. 2000;102:1564-1568.
112. Khaw B.A. The current role of infarct avid imaging. Semin Nucl Med. 1999;29:259-270.
113. Flotats A., Carrio I. Non-invasive in vivo imaging of myocardial apoptosis and necrosis. Eur J Nucl Med Mol Imaging. 2003;30:615-630.
114. Blankenberg F.G., Katsikis P.D., Tait J.F., et al. In vivo detection and imaging of phosphatidylserine expression during programmed cell death. Proc Natl Acad Sci U S A. 1998;95:6349-6354.
115. Ogasawara J., Watanabe-Fukunaga R., Adachi M., et al. Lethal effect of the anti-Fas antibody in mice. Nature. 1993;364:806-809.
116. Hofstra L., Liem I.H., Dumont E.A., et al. Visualisation of cell death in vivo in patients with acute myocardial infarction. Lancet. 2000;356:209-212.
117. Laguens R.P., Meckert P.M., Martino J.S., et al. Identification of programmed cell death (apoptosis) in situ by means of specific labeling of nuclear DNA fragments in heart biopsy samples during acute rejection episodes. J Heart Lung Transplant. 1996;15:911-918.
118. Narula J., Acio E.R., Narula N., et al. Annexin-V imaging for noninvasive detection of cardiac allograft rejection. Nat Med. 2001;7:1347-1352.
119. Tokita N., Hasegawa S., Tsujimura E., et al. Serial changes in 14C-deoxyglucose and 201Tl uptake in autoimmune myocarditis in rats. J Nucl Med. 2001;42:285-291.
120. Faust A., Wagner S., Law M.P., et al. The nonpeptidyl caspase binding radioligand (S)-1-(4-(2-[18F]Fluoroethoxy)-benzyl)-5-[1-(2-methoxymethylpyrrolidinyl)s ulfonyl]isatin ([18F]CbR) as potential positron emission tomography-compatible apoptosis imaging agent. Q J Nucl Med Mol Imaging. 2007;51:67-73.
121. Zhou D., Chu W., Rothfuss J., et al. Synthesis, radiolabeling, and in vivo evaluation of an 18F-labeled isatin analog for imaging caspase-3 activation in apoptosis. Bioorg Med Chem Lett. 2006;16:5041-5046.
122. Corbett J.R., Lewis M., Willerson J.T., et al. 99mTc-pyrophosphate imaging in patients with acute myocardial infarction: comparison of planar imaging with single-photon tomography with and without blood pool overlay. Circulation. 1984;69:1120-1128.
123. Orlandi C., Crane P.D., Edwards D.S., et al. Early scintigraphic detection of experimental myocardial infarction in dogs with technetium-99m-glucaric acid. J Nucl Med. 1991;32:263-268.
124. Johnson G.III, Okada C.C., Hocherman S.D., et al. (99m)Tc-glucarate imaging for the early detection of infarct in partially reperfused canine myocardium. Eur J Nucl Med Mol Imaging. 2006;33:319-328.
125. Narula J., Petrov A., Pak K.Y., et al. Very early noninvasive detection of acute experimental nonreperfused myocardial infarction with 99mTc-labeled glucarate. Circulation. 1997;95:1577-1584.
126. Mariani G., Villa G., Rossettin P.F., et al. Detection of acute myocardial infarction by 99mTc-labeled D-glucaric acid imaging in patients with acute chest pain. J Nucl Med. 1999;40:1832-1839.