CHAPTER 5 Lumbar Musculature
Anatomy and Function
Physiology
The myofibrils attach to the sarcolemma at two ends, which connects one cell to its neighbor in the fiber structure. The myofibrils are highly organized, aligning longitudinally within the sarcolemma, which itself is indented by a motor axon at its myoneural junction. By convention, the smallest contractile subunit within the myofibrils is called the sarcomere. The sarcomere is composed of smaller subunits called myofilaments. The myofilaments are organized longitudinally with alternating light and dark striations (hence skeletal muscles are known as “striated muscle”) when visualized microscopically. The myofilaments within the sarcomere (smallest contractile subunit) are composed predominantly of two protein varieties: myosin and actin.1
Under normal circumstances, contraction of striated muscle does not occur without a neural stimulus, whereas contraction of cardiac and most smooth muscle fibers can trigger adjacent fibers to contract without neural stimulation. The cellular mechanics of contractions are relatively simple: Actin filaments (occupying the light-colored I-band at rest) slide over the myosin filaments (found in the A-band and interdigitating with I-band at rest) until, with complete contraction, they completely overlap and eliminate the light H-band under microscopic visualization. The biochemical reactions are far more complex (Fig. 5–1). Contraction is initiated by release of acetylcholine at the myoneural junction, depolarizing the sarcolemma by changing its permeability to sodium and potassium ions. This sarcolemma stimulates release of calcium ions, sequestered in the sarcoplasmic reticulum, that bind to the troponin complex (C, T, and I). Calcium ions binding troponin induce a conformational change, which uncovers the binding portion of the actin filament. Myosin binds and unbinds actin, in concert with adenosine phosphate molecules (adenosine triphosphate [ATP] and adenosine diphosphate [ADP]), to induce the “racheting” of the myosin along the length of the actin filament.
A growing body of research shows that lactate is not a “dead-end metabolite,” and it is not the “mediator” of fatigue and inefficiency as widely published in the 1960s through the 1980s.2 On the contrary, current research implicates the hydrogen ion excess as the primary agent of diminished contractile power. The lactate ion may serve multiple roles in maintaining constant energy; recruiting new energy sources (gluconeogenesis); recruiting new vascularity (angiogenesis); and promoting a local cascade of healing, plasticity, and hyperplasia.2–4 A growing body of research based on the experimental work of Brooks,4 termed lactate shuttle theory, suggests that higher concentrations of lactic acid produced in the skeletal muscles stressed by exercise have significant increased benefit in remote tissue such as brain, liver, heart, peripheral nerves, and peripheral vasculature over baseline metabolism.2
The ATPase work of Engel5 in 1962 established a body of research showing the presence of distinctly different motor units within skeletal muscle. There are many myotype classification schemes based on histology, morphology, or function. In brief, the interaction between the type of myosin heavy chain (ATP binding site) and actin within individual sarcomeres is probably the greatest contributor to functional differences within myofibrils. The functional difference is related to the rate that the myosin heavy chains can repetitively bind ATP and release ADP under conditions of physiologic stress.6
Roughly divided, sarcomeres fall into one of three broad functional categories. Type I fibers have a slower “twitch” response with good fatigue resistance and lower tension development.7 Structurally, these groups of sarcomeres (known collectively as fibers) have rich capillary beds and high concentrations of mitochondrial enzymes with relatively low concentrations of glycogen and myosin ATPase. They seem ideally suited for aerobic activity with good fatigue resistance. These type I muscles predominate in areas that require aerobic or endurance demands. Type II muscle displays a fast twitch with good strength but relatively poor endurance compared with type I muscle fibers. Type II fibers can be subdivided further into type IIA, which still show a fast twitch response but a fatigue threshold between type I and type IIB, and type IIB, which show the fastest contractions, the highest tension development, and the most rapid onset of fatigue.7,8 Although other fiber subtypes continue to be identified, type I, type IIA, and type IIB show the major functional categories of voluntary skeletal muscles.7 Whether individual fibers have biochemical characteristics for high-intensity, short-duration contractile bursts or more sustained activity, each muscle is a heterogeneous, woven tapestry of all of the above-mentioned fiber subtypes. Any relative predominance of one particular fiber type is based on genetics, anatomic location of the muscle, demand on the muscle, age of the individual, nutrition, and multiple other external factors.9
Although the contribution to parental lineage to phenotypic expression may remain paramount in predicting functional potential, the actual fiber composition of muscle groups has great capacity for plasticity in response to environmental stress. Multiple studies show fiber conversion within major groups from type IIB to type IIA to be common.10 Other conversions, such as type I to either type IIA or type IIB, are less common and seen most often with denervation, immobilization, and profound deconditioning.8 There is emerging evidence of conversion of type II to type I in the electrical stimulation literature, but data remain sparse and confounded by the fact that type II muscle tends to atrophy with age. The relative absence of type II muscle may confound data trying to analyze conversion.11
A human infant is normally born with a full complement of muscle fibers that may continue to differentiate in childhood. Muscle fiber genesis seems to be biphasic. Myoblasts fuse to form fibrils at 8 to 10 weeks of gestation—primarily resulting in the formation of type I (slow-twitch) fibers. The second wave of fiber formation occurs at 15 to 18 weeks of gestation creating fast-twitch, type II fibers, which differentiate and change with early postpartum use.7 The growth and change in muscle size after adolescence seems to be due to increase in size of the fibers rather than increase in numbers (although controversial studies still contend that extreme muscle exertion may result in some new fiber development) that accompanies increased neuronal innervation and finer control of contractile force.
Although animal data suggest that hyperplasia and genesis of new muscle fibers may be possible with training and endogenous, hormonal feedback, these data have not been replicated in humans. Instead, increasing strength of contraction with training seems to be related not only to muscular factors, such as muscle size, fiber type, and fiber number, but also (and perhaps to a greater extent) to neural factors.12 The specific neural characteristics that change with training include frequency, extent, order, and synchrony of motor unit firing, with feedback to the spinal pyramidal tracts to control system efficiency—maximal force at minimal energy expenditure. Training, age, and certain anabolic hormones (either endogenous or exogenous) seem to exert the greatest effect on muscle plasticity—the characteristic rapid increase in strength and muscle diameter under the hormonal influence of puberty is a specific example.
Anatomy
The spine consists of a series of bilaterally symmetrical joints phylogenetically adapted for protection of the neural communications network linking brain to periphery.13 The critical role of the spine musculature in dynamically protecting and vitalizing these articulations with their passive ligamentous supports and accompanying neural transmission lines is infrequently acknowledged in biomechanical modeling. For this reason, a perspective based in evolutionary theory aids in understanding the complexity of lumbar spine musculature.14 From an evolutionary standpoint, it seems that all land-dwelling vertebrates (including mammals) evolved from ocean-dwelling cousins; in the ocean, gravitational force acted differently when contributing to function and form. Large paravertebral spine muscles likely developed to provide lateral (coronal plane) flexion-extension to propel bodies through water, as shown by the lateral tail motion of the fish. This form of locomotion was initially preserved in amphibians and land reptiles (even after the development of extremities), as seen in the Crocodylia species including the modern alligator and crocodile. Reptiles with legs (currently living) all propel themselves through lateral spinal motion in which propulsion is achieved by contracting spine muscles to create alternating coronal convexities on one side of the spine that allow the ipsilateral foreleg to move forward while the contralateral hindfoot (on the concave side) is brought closer to the contralateral foreleg in preparation for the next reciprocal lateral movement that repeats the motion-event.
Mammalian adaptations resulted in a 90-degree evolutionary shift to the sagittal spinal motion characteristic of all four-legged mammals (the platypus and echidna being partial exceptions), which presumably provides an advantage during land locomotion, allowing for explosive growth of the class Mammalia. Land mammals that subsequently retreated to the sea (e.g., whales, porpoises, seals) maintain the sagittal orientation of spinal locomotion resulting in the 90-degree rotation of the tail fluke (when compared with fish), even after adaptive pressure resulted in other changes to their extremities. The later adaptation of humans to full bipedal stance and locomotion presumably necessitated a lordotic lumbar spine, kyphotic thoracic spine, and lordotic cervical spine for balance and ambulation (Fig. 5–2).
It is further theorized that the lordotic curve converts lateral bending to produce torsion at the hips, adding propulsion efficiency to the balanced human gait that would be impossible without lumbar lordosis. Ambulation without lumbar lordosis leads to the shuffling strides of the upright apes, whose gait is clearly dissimilar to that of humans. Laboratory modeling strongly suggests that cocontraction of spinal and abdominal muscles is the primary generator of the curvilinear structure of the spine that sustains logarithmically more force than “straight” models of spine motion (>1200 N vs. 100 N). The theory holds that instantaneous, axial-rotational forces between segments in “straight-spine” models lead to rapid failure when the spine is progressively loaded.15
This model focuses on the dynamic contribution of spine muscles to create a compressive-stabilizing force through bony and ligamentous structures that withstands physiologic forces modeled in sagittal motion. Similar to taut “guy wires” or tensioned cables adding structural stability to allow gauzy tent material to withstand 90 mph winds, muscle tension is hypothesized by the “follower-load” theory to provide a stabilizing force (in at least one plane of motion—sagittal) that directs the force vector to pure compression of the motion segment, minimizing shear (shear force being implicated in degenerative cascade).16
Another unique human evolutionary adaptation for manipulation of objects near the ground from a bipedal stance involves the ability to bend efficiently from the waist in combination with squatting and hunkering. A stable “biomechanical chain,” transferring force efficiently from hands through arms, shoulder girdle, spine, pelvis, legs, and feet to make ground contact, is necessary (Fig. 5–3). In this functional concept, the muscle acts as a dynamic stabilizer of the biomechanical chain in several ways. During flexion-extension of the lumbar spine, the co-tensioning abdominal muscles at a distance (force plus lever arm) allows maintenance of a “balance point” at each individual motion segment. Next, coupled anterior and posterior forces have a net compressive (downward) force, which balances motion at the instantaneous axis of rotation for each motion segment—maintaining compression at the disc and minimizing angular change. The net muscle tension force downward serves to offset any other forces, maintaining the force vector perpendicular to the disc’s plane similar to the tent “guy-wires” explained previously.16,17 Muscles supply dynamic and static downward force to create a form that allows maximal load bearing (for bipedal carrying and lifting) capacity, while maintaining function that allows maximal efficiency of muscle energy output—maintaining a plumb line (not working against gravity to maintain posture), sharing tension bands to distribute loads, coupling forces when motion is required, and distributing load/work among the other osteoligamentous static structures.
These observations lead to multiple functional inferences, one of which is the tendency of the spine to “hang on its ligaments” in an efficient, muscle-sparing manner frequently observed in “stooped laborers.” Efficiency of motion is documented by the way most normal subjects make the universal unconscious choice of allowing spine flexion to precede hip flexion when bending forward at the waist, eventually resulting in an EMG “silent period” when the spine is fully flexed and stabilized by posterior ligaments.14,18,19
The small interconnecting vertebrae motion segments with the multiplanar motions of the three-joint complex produce difficulties when assigning specific uniaxial functions to individual groups of muscles. The erector spinae group of muscles are generally thought of as extensors of the spine. Functioning unilaterally, they may also be powerful abductors or lateral stabilizers (assisting with locomotion) and have been shown to function to some extent in spine derotation.18 Similarly, lateral abdominal musculature (internal and external oblique and transversus abdominis) may act as spine flexors and extensors (working through the lumbosacral fascia).14 The “girdling” abdominal muscles are also powerful spine rotators and assist in abduction and lateral stabilization.20 As noted previously in this chapter, the force-coupling of spinal, pelvic, and abdominal muscles acting in concert increases compressive load through the disc and vertebral bodies to minimize shear force and imbalance axially across the three-joint complex.
In describing the gross anatomy of the spinal muscular functional unit, the extreme importance of the posterior stabilizing structures cannot be overemphasized in spinal functional integrity. The intrinsic lumbar musculature is only a part of the functional unit. The lumbodorsal fascia, interspinous ligaments, and facet joint capsules are crucial structures providing constraint and fulcrum points.21 There is growing appreciation among some surgeons to maintain the integrity of these collagenous and elastic structures, which may decrease adjacent segment shear, dysfunction, or instability.
Mammals as a class share many characteristics, including those of the spine musculature, that may account for the evolutionary success of the class. The most superficial layers of spine musculature, extensions of the functional unit of the shoulder girdle, include muscles such as the serratus posterior and latissimus dorsi. In contrast to other spinal musculature, the modified proximal function of these muscles is matched by innervation from the proximal spinal cord. The true lumbar spinal muscles, by contrast, have segmental innervations that arise from the posterior rami of the contiguous spinal nerves (the same nerves that perceive proprioceptive input from facet capsules, posterior ligaments, and peripheral anulus). Although true spinal muscles function together, their most characteristic differentiating factor is span length. The deepest muscles, such as the interspinalis, span only a single segment. The most superficial muscles may traverse a large portion of the entire spinal column. Controlled, coordinated action of individual vertebrae is a critical part of spine function, whereas loss of appropriate musculoligamentous control may contribute to various pathologic syndromes, such as segmental instability, segmental rigidity, facet syndromes, and perhaps even discogenic pain.22
Musculature of the Lumbar Spinal Functional Unit
Intrinsic Muscles
Erector Spinae
The erector spinae is a large and superficial muscle that lies just deep to the lumbodorsal fascia and arises from an aponeurosis on the sacrum, iliac crest, and thoracolumbar spinous processes.13 The muscle mass is poorly differentiated, but divides into three sections in the upper lumbar area: (1) The iliocostalis is most lateral and inserts into the angles of the rib; (2) the longissimus, the intermediate column, inserts into the tips of the spinous processes of thoracic and cervical vertebrae; (3) the spinalis is most medial and inserts into spinous processes of the cervical and thoracic vertebrae (Fig. 5–4). The innervation of all the lumbar paraspinal muscles is from the dorsal rami of the nerve root as it exits at the most approximate level and overlaps proximally and distally along the muscle length.
Multifidi
Multifidi are a series of small muscles, best developed in the lumbar spine, that originate on the mammillary processes of the superior facets and run upward and medially for two to four segments, inserting on the spinous processes (Fig. 5–5). This orientation produces greater capacity for rotation and abduction, in addition to extension. They also share a multilevel innervation (similar to the erector spinae bundle or group) where function is maintained even with injury to the proximate dorsal rami.
Quadratus Lumborum
The quadratus lumborum is the most lateral of the lumbar muscles (see Fig. 5–4); it originates on the iliac crest and iliolumbar ligament and runs obliquely to insert into the lowest rib and transverse processes of the upper four lumbar vertebrae. Its orientation provides strong abduction motor and stabilizer properties. The innervation is from the ventral rami of T12-L1-L2-L3 roots.
Psoas and Iliacus Muscles
The psoas major, although usually thought of primarily as a hip flexor, has a direct effect on the vertebral column because it originates bilaterally from the vertebral bodies and posterior aspects of the transverse processes, providing the only intrinsic spinal muscle acting anterior to the sagittal axis. Yet, paradoxically, the psoas is usually an intersegmental extensor in the mid-lumbar spine, even as it flexes at the lumbosacral junction in the process of increasing the lumbar lordosis. It is an important spine stabilizer in sitting and standing.23 Acting asymmetrically, the psoas may produce abduction or abduction-resistance to maintain coronal balance. Pathophysiologically, low back or pelvic pain may result from contracture or spasm of the iliopsoas producing combined hip and lumbosacral junction flexion. As in other situations, appropriately targeted stretches, followed by strengthening, may produce dramatic improvement.
Extrinsic Muscles
Abdominal Musculature
There are four important abdominal girdling muscles in spine function.24 The rectus abdominis is primarily a flexor, spanning the anterior abdomen from its origin on the pubic crest to its insertion on the anterior rib cage between the fifth and seventh ribs. The obliquely oriented abdominal muscles are, from superficial to deep, the external oblique, internal oblique, and transversalis abdominis. They all may act to produce rotation or abduction and assist flexion and extension under different circumstances.14
The fibers of the external oblique run in an anteroinferior direction from attachments on the lower eight ribs to insert along the anterior rectus sheath and anterior wall of the iliac crest (Fig. 5–6). The external oblique fibers are almost perpendicular in direction to those of the internal oblique fibers. This muscle courses transversely only in its lowermost portion, with most of the muscle running anteriorly and proximally from its origins on the lumbodorsal fascia and anterior two thirds of the iliac crest. It inserts on the lower three ribs and rectus sheath anteriorly. The transversalis abdominis, the deepest muscle of the group, runs transversely like a horizontal girdle from the lumbodorsal fascia, anterior iliac crest, and inner surface of the lower six ribs. The main mass of the muscle inserts into the linea alba in the midline. In the act of flexion, it is probable that the abdominal muscles act not only to create a ventral moment, but also to stabilize the spine posteriorly through their action on the lumbodorsal fascia. The innervation of the abdominal muscles is shared via intercostal nerves from root levels T7-T12. The thoracic nature of the innervation means that these muscles are spared from radicular-type injuries.
Gluteal Muscles
The large muscles of the buttocks, chiefly the gluteus maximus, gluteus medius, and gluteus minimus, act variously as hip extensors and abductors. As such, they act as motors to the spinal “crane” in forward bending and twisting movements. They also provide the “spinal engine” for locomotion (Fig. 5–7).13,14 The gluteus maximus receives innervation via the inferior gluteal nerve and is primarily an S1 muscle, although it receives contributions from L5 and S2 root levels. The superior gluteal nerve innervates the gluteus medius primarily with L5 contribution. Although it receives contributions from L4 and S1 root segments, it is often an important “internal verifier” of a true L5 radiculopathy when combined with clinical or electrodiagnostic abnormalities in distal L5 muscles.
Electrophysiology
EMG employed to analyze normal function, as opposed to pathologic conditions, of the spine musculature is less well understood. There has not been a concerted research effort to document the natural history of adaptive electrodiagnostic findings as patients evolve from symptomatic to asymptomatic. This section discusses the clinical utility and limitations of electrodiagnostics further. In assessing low back pain without corresponding neurologic sequelae in the extremities, EMG and nerve conduction velocity remain investigational. Biomechanical studies have often relied on use of the raw, integrated EMG or root mean square EMG signals to estimate muscle loads in studies involving lifting performance in normal subjects. Trunk stabilization and initiation of motion are two particular patterns of EMG activity that have been identified in trunk movements.25–27 Different movements recruit muscles in varying patterns of activity. Intrinsic spine musculature supplies a net sum of movement using various lever arms to initiate and arrest motion by combining concentric and eccentric contraction in either movement or maintenance of posture.
Longissimus and other paravertebral muscles are frequently silent in the “flexion-relaxation” position, in which the maximally flexed spine is “hanging on its posterior ligaments.” It is also relatively quiet in gentle extension of the spine, but with full extension, lateral bend, or torsion, the role of the intrinsic musculature is as a “balancer” of spinal motion—shown by its prominent activity.26 Subsequently, it was found that loading of the flexed spine in the posture normally producing EMG silence leads to an increase in myoelectric activity in the intrinsic spine musculature proportional to the load applied. This proportion of increased EMG and muscle activity is similar to the changes seen in loading the spine in the upright position.28 Presumably, these increasing loads stress and stretch the posterior ligamentous structures sufficiently to require compensatory muscle firing. The multifidus and rotatores muscles have similar activity in sagittal plane movements. They are active in rotation to the contralateral side, however, in conjunction with bilateral abdominal muscular contraction.20
These muscles also achieve flexion-relaxation silence when the spine is in the ligamentous support phase. Early investigators discovered that the slouching or full flexion seated posture (often considered “bad posture”) is, in reality, quite comfortable for prolonged periods and that EMG silence is generally maintained in the erector spinae.29 Issues of posture, movement, load, and speed have usually been studied through surface EMG measurements.30 This “silent period” is also known as the flexion-relaxation phenomenon.31 The phenomenon represents an EMG pattern seen in most normal subjects but frequently absent in subjects with chronic low back pain. These patients show elevated muscle activity during full voluntary trunk flexion and fail to achieve flexion-relaxation.32–36 The phenomenon is noted with needle and surface EMG measurements, which has led many researchers to use surface EMG in studying the phenomenon.37
More recent research suggests that the hypothesis of a dichotomous pattern (muscles either “on” or “off”) is likely misleading. Physiologically, lumbar muscles show electrical activity because they are contracting, and the degree of electrical activity is roughly proportional to the rate and amplitude of muscle fiber firing. Lumbar muscle activity is almost never “completely silent” during trunk flexion maneuvers. More recent descriptions of the phenomenon suggest a significant contractile force associated with high surface EMG activity during flexion, followed by a relaxation phase associated with low surface EMG activity.38,39
Several researchers have also proposed quantitative formulas for defining the presence or absence of flexion-relaxation.36,40,41 More recent work has compared inclinometric range of motion and surface EMG measures, with several interesting findings.19 Using this quantified approach, almost all normal subjects can achieve flexion-relaxation, even if they lack completely normal spine motion. Even in patients with chronic low back pain who are symptomatic and completely disabled, approximately 30% can achieve flexion-relaxation before rehabilitation. After a functional restoration program that stressed improved lumbar mobility with pain management techniques aimed at the lumbar musculature, however, 94% of the patients completing treatment achieved flexion-relaxation. Larger studies performed subsequently show that quantified lumbar flexion-relaxation phenomenon can be an objective tool for measuring improvement in a functional restoration program, correlated to improvements in lumbar range of motion measurements.42 Almost all the improvement in achieving flexion-relaxation is directly attributable to a component of the interdisciplinary program that involves surface EMG biofeedback involving surface EMG–assisted stretching training.43
Contractions of the abdominal musculature, particularly muscles attaching to the lumbosacral fascia, are also important in maintaining flexed postures and initiating extension. The lateral pull on the lumbodorsal fascia has two effects: creating a tightening of the craniocaudal dimension in the lumbar spine (“guy-wire model” discussed earlier) and “encapsulating” the intrinsic spine musculature to provide greater efficiency. In so doing, the abdominal mechanism also serves as a force-coupling mechanism: eccentrically controlling extension of the flexed spine while resisting flexion loads.14
Some groups have tried to correlate functional tasks and training, EMG data, and computed tomography (CT) and magnetic resonance imaging (MRI).44 Small studies have shown correlations between postoperative strength deficits, imaging of muscle atrophy (decreased cross-sectional area or fatty infiltration), and changes in EMG characteristics.18,45,46 CT seems to provide reliable measurements of paraspinal muscle cross-sectional area and density in normal subjects and patients with chronic low back pain.47–49 Although some early pilot work at several institutions is intriguing, serial imaging remains cost prohibitive and is of little value for altering treatment outcomes. More recently, needle EMG sampling of the multifidus has been described in the diagnosis of lumbar spinal stenosis.
Trunk Muscle Strength
The obvious relationship between extremity joints and strength of the contiguous musculature in athletic and pathologic (traumatic, arthritic, or deconditioned) situations has stimulated many investigators to study similar relationships in the spine.29,50 Early investigators were limited to the use of cable tensiometers and isometric and isotonic machines. Work began 30 years ago using individually modified isokinetic dynamometers in various positions.51–53 Later technology provided computerized isokinetic devices for separately measuring isolated lumbar trunk strength in the sagittal and axial planes concentrically and eccentrically that also allowed measurements isometrically and isotonically.20,41,54–56
Isokinetics has been deemed a safe way to measure muscular output.57–59 Studies have shown a diversity of normative data owing to factors such as different protocols, positioning (reclined, sitting, or standing), instrument, study samples, gravity correction, and axis of rotation. The normative data are specific to instrumentation, protocol, and positioning.60–63 The development and availability of isokinetic dynamometers or attachments specifically for back testing increased beginning around 1980. The number of manufacturers of isokinetic dynamometers available to test the back has decreased since then as a result of a combination of managed care reimbursement issues and lack of training for physical or occupational therapists likely to use such devices. At the present time, only Biodex (Shirley, NY), CSMi (formerly Cybex; Stoughton, MA), and Technogym (Gambettola, Italy) remain, limiting the development in the field of quantitative functional measurement of the spine (Fig. 5–8).
Cady and colleagues64 showed a relationship between physical fitness and back injury rates that has been confirmed through isometric lifting testing in several industrial environments. Blay and colleagues65 supported the use of isokinetic measurements for the assessment of trunk muscle strength in scoliotic patients. Results indicate that the reliability of trunk testing was satisfactory (Fig. 5–9). These results are in accordance with other studies of trunk testing relating to healthy subjects and patients with low back pain.66 Although joint range of motion seems to be an independent variable in trunk function, muscular factors such as trunk strength, endurance, and neuromuscular coordination seem to be critical factors in maintaining the integrity of the lumbar spine. Tests of isokinetic trunk strength in the sagittal plane have revealed a typical gaussian distribution of strength in the normal population. Normalizing strength by body weight narrows the width of the distribution curve. Using other normalizing factors, men seem to be 10% to 20% stronger than women and have greater ability to sustain strength at high speeds. The variety of test methods used to examine trunk muscles makes it difficult to compare results. The assessment requires an understanding of the force relationship throughout the defined range of motion (work).67
Another challenge is the absence of a contralateral measurement within the same person to provide a “gold standard.” Comparison with a normative database stratified by age (past the 5th decade), weight, and gender has emerged as the standard for quantifying strength. Patient inhibition by pain or reduced voluntary effort is often a major factor in low torque output (Fig. 5–10), a pattern that had been identified in sports medicine testing of limbs but not as well documented in patients with chronic low back pain. Age through the 5th decade does not seem to be a critical variable.
Similar findings are noted when measuring isolated axial strength.20,68,69 Ability to generate trunk rotational torques within the same individual is generally symmetrical, although there is a trend toward slightly greater strength rotating to the dominant side in men, which may be due to training in pulling activities. There seems to be a greater tolerance for applying loads at high speeds in the axial plane. This tolerance may be due to the greater fast-twitch fiber type composition of the lateral abdominal musculature, which is primarily responsible for axial plane lumbar spine movements. Rotational strength depends on the direction; for men and women the force produced from the rotated position toward the neutral position is stronger than the neutral position rotating outward.70
In the pathologic state, significant decrements of muscle strength are frequently noted. Patients with chronic back pain show a selective loss of extensor strength compared with flexors and an inability to maintain strength at high speeds.59,71–74 In rotation, there is also a substantial decrement in strength, but it seems to be relatively symmetrical in the chronic state and less subject to high-speed variation.54 Although most early work was based on peak torque measurements, advances in computerization made measurements of work performed, power consumed, and curve analysis possible, while allowing variability determination to assess “effort.” Because only maximal muscular effort is truly reproducible, variability of curve shape and height becomes a potential measure of effort. In the absence of visual feedback of trunk muscle function to the clinician, these measures become exceedingly important in documenting optimal functional capacity and effort.75,76 Some controversy exists regarding clinical utility and discriminating power of trunk strength testing, partly because of normal human variability and partly because of unrecognized sources of error related to testing procedures.59,73,77 The discriminating power of a test depends not only on the ability to distinguish the “normal” from the pathologic state, but also on distinguishing prerehabilitation and postrehabilitation performance. Many such longitudinal studies have been performed, including some that correlate spinal strength performance to imaging (e.g., CT and MRI) findings.18,45,78–81
By contrast, supernormal or athletic individuals who have been studied (Fig. 5–11), such as female gymnasts, male soccer players, male tennis players, and male wrestlers, seem to exceed mean torque-to-body weight strength ratios for the normal population by 15% to 40%.82 In contrast to pathologic and normal populations, supernormal or athletic individuals show almost no “high-speed drop-off”—that is, decreased torque output at high speeds. They also maintain a very stable ratio of extensor to flexor strength (well-balanced, efficient use of coupled forces).
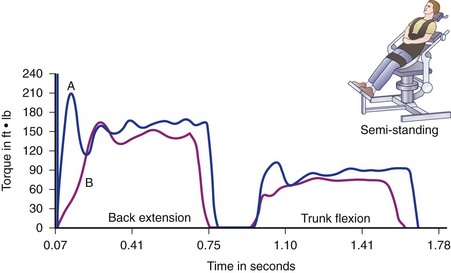
FIGURE 5–11 Normal force production at two different speeds for an athlete. A, 60 degrees/sec. B, 120 degrees/sec.
The factors that contribute to decreased strength in the pathologic state are not entirely understood. Although muscle atrophy undoubtedly occurs with prolonged disuse and deconditioning, pain may inhibit neuromuscular function through nociceptive reflex feedback mechanisms. Similarly, various psychosocially induced phenomena, such as anxiety, fear of reinjury, or depression, may unconsciously attenuate effort, producing submaximal measurements. At this time, techniques for assessing subject motivation do not seem to be available, although curve variability and ratio of work to peak torque seem to be promising tools for identifying attempts to produce submaximal output consciously.76,83
Myofascial Pain
A highly used but poorly understood treatment for lumbar myofascial or “back muscle pain” is “trigger point” management. Trigger points themselves are fairly well described, hyperirritable, isolated, focal areas in a “taut band” of multiple fibers of skeletal muscle that produce sustained contraction that fails to relax or release fully, which creates local and referred pain in discrete, predetermined patterns.84 Although numerous authors have described the diagnosis and treatment of trigger points, many others have questioned the interphysician reliability of judging trigger points and the more slippery generalized diagnosis of myofascial pain.85–88 Although by no means definitive, a growing number of physicians diagnose the widespread, painful summation of multiple areas of hyperirritability nonspecifically as myofascial pain or fibromyalgia—which is treated partially with trigger point injection and pharmacologic intervention.
Although trigger points are a clinical diagnosis, some academicians believe that the presence of a local twitch response (identified most reliably with EMG) is a prerequisite to treatment.84,85 Nevertheless, many patients find relief from interventions aimed at modifying trigger points, even if present in multiple body regions. Treatments directed toward trigger points are widespread among many different physician fields and alternative medicine specialists. Although many practitioners have a favorite “needling” technique, multiple studies have found no statistical difference between “dry needling,” saline injection, or medication.89 The preeminence of needle injection as the most effective way to inactivate a trigger point has been questioned in several published articles.90,91 Other modalities for refractory myofascial or trigger point pain include ultrasound, manipulation, massage, acupressure, acupuncture, and “spray and stretch” techniques.
Within the last decade, use of botulinum toxin (Botox) has become popular, first to inactivate refractory trigger points and then more generally for use in muscle spasticity associated with back pain. Botulinum toxin, one of the most potent biologic poisons known, is created by a gram-positive, rod-shaped bacillus, Clostridium botulinum, first identified in the 1820s. The toxin is a heat-labile, zinc-dependent, metalloprotease polypeptide composed of a heavy chain and a light chain about 150 kDa in size (too large to cross the blood-brain barrier) that denatures at 80° C.92–94 There are currently eight classified toxin types: A, B, C1, C2, D, E, F, and G. The presynaptic, cholinergic neuromuscular junctions are the target of botulinum toxin. The toxin inhibits the release of acetylcholine, which results in blockage of muscle activation and paralysis.95,96 The toxin targets zinc-dependent endoproteases of the SNARE complex—SNAP-25, synaptobrevin II, and syntaxin I—each involved in the packaging and release of acetylcholine at the postsynaptic nerve terminal.97 Each toxin type (A to G) acts slightly differently on the three SNARE proteins, leading to speculation, without clinical evidence, that different toxins may have different clinical uses and safety profiles.
Muscle-Sparing Surgery
Lumbar surgery has classically involved extensive dissection of the posterior muscle, fascia, ligamentous structures, and occasionally joints that is even more extensive when fusion (with or without instrumentation) is attempted. Additionally, unintended consequences of denervation, compression, retraction, and vascular injury have led to other biomechanical sequelae.18,98 Decreased morbidity by sparing vulnerable structures may serve to maintain mobility and function, improving outcomes.
The posterior lateral approach first described by Cloward99 in 1953 has been controversial.100 The improved tubular retractor combined with fluoroscopy and improved implantable devices have allowed for a repopularization of this approach, although denervation and devascularization of the deep muscles is still a likely possibility. The more lateral approach first described in 1968 by Wiltse and colleagues101 for fusion allows paraspinal muscles to be bluntly divided along their aponeurosis sparing the muscle, fascia, and some of the ligamentous structures. Adequate decompression of far lateral or foraminal disc herniations can be achieved, but more centrally located pathology is difficult to access from this approach. Good fusion rates are reportedly achieved with marginally increased operative time and conflicting length of stay comparisons as a tradeoff for “sparing” devascularization and denervation injury to muscle.102 Multiple sources advocate percutaneous or open supplementary instrumentation to the contralateral side to achieve a consistently high fusion rate.
The anterior transperitoneal approach has been described since the 1930s in various iterations.103 A spine surgeon can achieve (indirect) decompression, stabilization, fusion (at a variable rate), and now motion preservation through this approach. Often these surgeries lose the benefit of posterior muscle preservation when surgeons later elect for additional posterior stabilization. Additionally, retraction can damage abdominal and anterior lumbar musculature that play a key role in maintenance of proper spine balance. The anterior approach has been modified to go retroperitoneally with laparoscopic devices that allow perceived (unconfirmed) benefit of splitting of the abdominal musculature to allow for more rapid postoperative healing and less perturbation of the abdominal viscera but often at the cost of crossing and possibly injuring the psoas muscle, which may have a very important role in lumbar pelvic coordination of functional tasks. Keeping in mind the advantages of protecting lumbar musculature and maintaining mobility, there is hope that the functional outcomes of spine specialists will continue to improve.
Summary
Key Points
1 Brooks GA. Intra- and extra-cellular lactate shuttles. Med Sci Sports Exerc. 2003;32:790-799.
2 Antonio JA, Gonyea WJ. Skeletal muscle fiber hyperplasia. Med Sci Sports Exerc. 1993;25:1333-1345.
3 Patwardhan AG, Havey RM, Carandang G, et al. Effect of compressive follower preload on the flexion-extension response of the human lumbar spine. J Orthop Res. 2003;21:540-546.
4 Mayer T, Vanharanta H, Gatchel R, et al. Comparison of CT scan muscle measurements and isokinetic trunk strength in postoperative patients. Spine. 1989;14:33-36.
5 Neblett R, Mayer T, Gatchel R, et al. Quantifying the lumbar flexion-relaxation phenomenon: Theory, normative data and clinical applications. Spine. 2003;28:1435-1446.
6 Newton M, Waddell G. Trunk strength testing with iso-machines: I. Review of a decade of scientific evidence. Spine. 1993;18:801-811.
7 Styf JR, Willen J. The effects of external compression by three different retractors on pressure in the erector spine muscles during and after posterior lumbar spine surgery in humans. Spine. 1998;23:354-358.
8 Wiltse LL, Spencer CW. New uses and refinements of the paraspinal approach to the lumbar spine. Spine. 1988;13:696-706.
1 McComas A. Neuromuscular Function and Disorders. Boston: Butterworth; 1977.
2 Gladden LB. Lactate metabolism: A new paradigm for the third millennium. J Physiol. 2004;558:5-30.
3 Favero T, Zable AC, Bowman M, et al. Metabolic end products inhibit sarcoplasmic reticulum Ca2+ release and [3H]ryanodine binding. J Appl Physiol. 1995;78:1665-1672.
4 Brooks GA. Intra- and extra-cellular lactate shuttles. Med Sci Sports Exerc. 2003;32:790-799.
5 Engel WK. The essentiality of histo- and cytochemical studies of skeletal muscle in the investigation of neuromuscular disease. Neurology. 1962;12:778-784.
6 Brook MH, Engel WK. The histographic analysis of human muscle biopsies with regard to fiber types: Adult male and female. Neurology. 1969;19:221-233.
7 Staron R. Human skeletal muscle fiber types: Delineation, development, and distribution. Can J Appl Physiol. 1997;22:307-327.
8 Scott W, Stevens J, Binder-Macleod SA. Human skeletal muscle fiber type classifications. Phys Ther. 2001;81:1810-1816.
9 Antonio JA, Gonyea WJ. Skeletal muscle fiber hyperplasia. Med Sci Sports Exerc. 1993;25:1333-1345.
10 Staron RS, Malicky ES, Leonardi MJ, et al. Muscle hypertrophy and fast fiber type conversions in heavy resistance-trained women. Eur J Appl Physiol Occup Physiol. 1990;60:71-79.
11 Roy RR, Talmadge RJ, Hodgson J, et al. Differential response of fast hindlimb extensor and flexor muscles to exercise in adult spinalized cats. Muscle Nerve. 1999;22:230-241.
12 McArdle WD, Katch FI, Katch VL. Essentials of Exercise Physiology. Philadelphia: Lea & Febiger; 1994.
13 Hollinshead W. Anatomy for Surgeons, 3rd ed. Hagerstown, MD: Harper & Row; 1982.
14 Gracovetsky S, Farfan H. The optimum spine. Spine. 1986;11:543-573.
15 Patwardhan AG, Havey R, Meade K, et al. A follower load increases the load-carrying capacity of the lumbar spine in compression. Spine. 1999;24:1003-1009.
16 Stanley SK, Ghanayem A, Voronov L, et al. Flexion-extension response of the thoracolumbar spine under compressive follower preload. Spine. 2004;29:E510-E514.
17 Patwardhan AG, Havey RM, Carandang G, et al. Effect of compressive follower preload on the flexion-extension response of the human lumbar spine. J Orthop Res. 2003;21:540-546.
18 Mayer T, Vanharanta H, Gatchel R, et al. Comparison of CT scan muscle measurements and isokinetic trunk strength in postoperative patients. Spine. 1989;14:33-36.
19 Nachemson A. The possible importance of the psoas muscle for stabilization of the lumbar spine. Acta Orthop Scand. 1968;39:47-57.
20 Mayer T, Smith S, Kondraske G, et al. Quantification of lumbar function: III. Preliminary data on isokinetic torso rotation testing with myoelectric spectral analysis in normal and low back pain subjects. Spine. 1985;10:912-920.
21 Bogduk N, Macintosh J. The applied anatomy of the thoracolumbar fascia. Spine. 1984;9:165-170.
22 Macintosh J, Bogduk N, Gracovetsky S. The biomechanics of the thoracolumbar fascia. Clin Biomech. 1987;2:78-83.
23 Mayer T, Robinson R, Pegues P, et al. Lumbar segmental rigidity: Can its identification with facet injections and stretching exercises be useful? Arch Phys Med Rehabil. 2000;81:1143-1150.
24 Tesh K, Dunn J, Evans J. The abdominal muscles and vertebral stability. Spine. 1987;12:501-508.
25 Basmajian J. Muscles Alive: Their Functions Revealed by Electromyography, 4th ed. Baltimore: Williams & Wilkins; 1978.
26 Floyd W, Silver P. The function of the erector spinae muscles in certain movements and postures in man. J Physiol (Lond). 1955;129:184-203.
27 Morris J, Benner G, Lucas D. An electromyographic study of the intrinsic muscles of the back in man. J Anat. 1962;196:509-512.
28 Neblett R, Mayer T, Gatchel R, et al. Quantifying the lumbar flexion-relaxation phenomenon: Theory, normative data and clinical applications. Spine. 2003;28:1435-1446.
29 Flint M. Effect of increasing back and abdominal muscle strength on low back pain. Res Q. 1955;29:160-171.
30 Sarti M, Lison J, Monfort M, et al. Response of the flexion-relaxation phenomenon relative to the lumbar motion to load and speed. Spine. 2001;26:E421-E426.
31 Floyd W, Silver P. The function of the erector spinae muscles in flexion of the trunk. Lancet. 1951:133-143.
32 Ahern D, Follick M, Council J, et al. Comparison of lumbar paravertebral EMG patterns in chronic low back pain patients and non-patient controls. Pain. 1988;34:153-160.
33 Golding JS. Electromyography of the erector spinae in low back pain. Postgrad Med J. 1951;28:401-406.
34 Paquet N, Malouin F, Richards C. Hip-spine movement interaction and muscle activation patterns during sagittal trunk movements in low back patients. Spine. 1994;19:596-603.
35 Sihvonen T. Averaged (RMS) surface EMG in testing back function. Electromyogr Clin Neurophysiol. 1988;28:335-339.
36 Wolf SL, Basmajian JV, Russe CTC, et al. Normative data on low back mobility and activity levels: Implications for neuromuscular reeducation. Am J Phys Med. 1979;58:217-229.
37 Sihvonen T. Flexion-relaxation of the hamstring muscles during lumbar-pelvic rhythm. Arch Phys Med Rehabil. 1977;78:486-490.
38 Ng J, Vaughan K, Richardson C, et al. Range of motion and lordosis of the lumbar spine: Reliability of measurement and normative values. Spine. 2001;26:53-60.
39 Triano JJ, Schultz AB. Correlation of objective measure of trunk motion and muscle function with low back disability ratings. Spine. 1987;12:561-565.
40 Haig A, Weismann G, Haugh L, et al. Prospective evidence for change in paraspinal muscle activity after herniated nucleus pulposus. Spine. 1993;18:926-930.
41 Mayer T, Gatchel R. Functional Restoration for Spinal Disorders: The Sports Medicine Approach. Philadelphia: Lea & Febiger; 1988.
42 Mayer T, Neblett R, Brede E, et al. The quantified lumbar flexion-relaxation phenomenon (QLFRP) is a useful measurement of improvement in a functional restoration program. Spine. 2009;34:2458-2465.
43 Neblett R, Mayer T, Brede E, et al: Effectiveness of a clinical protocol for correcting abnormal lumbar flexion-relaxation in chronic lumbar pain. Spine. In press.
44 Mooney V, Gulick J, Perlman M, et al. Relationships between myoelectric activity, strength, and MRI of lumbar extensor muscles in back pain patients and normal subjects. J Spinal Disord. 1997;10:348-356.
45 Flicker P, Fleckenstein J, Ferry K, et al. Lumbar muscle usage in chronic low back pain: MRI evaluation. Spine. 1993;18:582-586.
46 Yoshihara K, Nakayama Y, Fujii N, et al. Atrophy of the multifidus muscle in patients with lumbar disc herniation: Histochemical and electromyographic study. Orthopedics. 2003;26:493-495.
47 Cooper RSt, Clair Forbes W, Jayson M. Radiographic demonstration of paraspinal muscle wasting in patients with chronic low back pain. Br J Rheumatol. 1992;31:389-394.
48 Damneels L, Vanderstraeten G, Gambier D, et al. CT imaging of trunk muscles in chronic low back pain patients and healthy control subjects. Eur Spine J. 2000;9:266-272.
49 Keller A, Gunderson R, Reikeras O, et al. Reliability of computed tomography measurements of paraspinal muscle cross-sectional area and density in patients with chronic low back pain. Spine. 2003;28:1455-1460.
50 Mayer L, Greenberg B. Measurement of the strength of trunk muscles. J Bone Joint Surg. 1942;24:842-856.
51 Langrana N, Lee C. Isokinetic evaluation of trunk muscles. Spine. 1984;9:171-175.
52 Smidt G, Herring T, Amundsen L, et al. Assessment of abdominal and back extensor function: A quantitative approach and results for chronic low-back patients. Spine. 1983;8:211-219.
53 Suzuki N, Endo S. A quantitative study of trunk muscle strength and fatigability in the low-back pain syndrome. Spine. 1983;8:69-74.
54 Mayer T, Smith S, Keeley J, et al. Quantification of lumbar function: II. Sagittal plane trunk strength in chronic low back pain patients. Spine. 1985;10:765-772.
55 Smith S, Mayer T, Gatchel R, et al. Quantification of lumbar function: I. Isometric and multispeed isokinetic trunk strength measures in sagittal and axial planes in normal subjects. Spine. 1985;10:757-764.
56 Mayer T, Gatchel R, Betancur J, et al. Lumbar trunk muscle endurance measurement: Isometric contrasted to isokinetic testing in normal subjects. Spine. 1995;20:920-927.
57 Dueker I, Ritchie S, Knox T, Rose S. Isokinetic trunk testing and employment. ACOEM. 1994;46:42-48.
58 Dvir Z. lsokinetics: Muscle Testing, Interpretation and Clinical Applications, 2nd ed. Philadelphia: Churchill Livingstone; 2004.
59 Newton M, Thow N, Somerville D, et al. Trunk strength testing with iso-machines: II. Experimental evaluation of the Cybex II back testing system in normal subjects and patients with chronic low back pain. Spine. 1993;7:812-824.
60 Benjamin S, Flood J, Bechtel R. Isokinetic testing prior to and following anterior lumbar interbody fusion surgery. A pilot study. IES. 2005;13:159-162.
61 Dervisevica E, Hadzica V, Burger H. Reproducibility of trunk isokinetic strength findings in healthy individuals. IES. 2007;15:99-109.
62 Skrzek A, Anwajler J, Mraz M, et al. Evaluation of force-speed parameters of the trunk muscles in idiopathic scoliosis. IES. 2003;11:197-203.
63 Palmer K. Reliability and typical isokinetic values as measured by the Biodex. IES. 1994;4:20-29.
64 Cady L, Bischoff D, O’Connell E, et al. Strength and fitness and subsequent back injuries in firefighters. J Occup Med. 1979;21:269-272.
65 Blay G, Atamaz F, Biot B, et al. Isokinetic findings in scoliosis: Their relationship to clinical measurements and reliability studies. IES. 2007;15:23-28.
66 Keller A, Hellesnes J, Brox J. Reliability of the isokinetic trunk extensor test, Biering-Sorensen test, and Astrand bicycle test. Spine. 2001;26:771-777.
67 Andersson E, Oddsson L, Grundstrom H, et al. The role of the psoas and iliacus muscles for stability and movement of the lumbar spine, pelvis and hip. Scand J Med Sci Sports. 1995;5:10-16.
68 Kumar S, Dufresne R, Schoor T. Human trunk strength profile in lateral flexion and axial rotation. Spine. 1995;20:169-177.
69 Ng J, Richardson C, Parnianpour M, et al. EMG activity of trunk muscles and torque output during isometric axial rotation exertion: a comparison between back pain patients and matched controls. J Orthop Res. 2002;20:112-121.
70 McIntire K, Asher M, Burton D, et al. Development of a protocol for isometric trunk rotational strength testing and strength asymmetry assessment. IES. 2007;15:183-194.
71 Beimborn D, Morrissey M. A review of the literature related to trunk muscle performance. Spine. 1988;13:655-660.
72 Malchaire J, Masset D. Isometric and dynamic performances of the trunk and associated factors. Spine. 1995;20:1649-1656.
73 Newton M, Waddell G. Trunk strength testing with iso-machines: I. Review of a decade of scientific evidence. Spine. 1993;7:801-811.
74 Sapega A. Current concepts review: Muscle performance evaluation in orthopaedic practice. J Bone Joint Surg Am. 1990;72:1562-1574.
75 Dvir Z, Keating J. Identifying feigned isokinetic trunk extension effort in normal subjects: An efficiency study of the DEC. Spine. 2001;26:1046-1051.
76 Hazard R, Reid S, Fenwick J, et al. Isokinetic trunk and lifting strength measurements: Variability as an indicator of effort. Spine. 1988;13:54-57.
77 Mooney V, Andersson G. Controversies: Trunk strength testing in patient evaluation and treatment. Spine. 1994;19:2483-2485.
78 Brady S, Mayer T, Gatchel R. Physical progress and residual impairment quantification after functional restoration: II. Isokinetic trunk strength. Spine. 1994;18:395-400.
79 Curtis L, Mayer T, Gatchel R. Physical progress and residual impairment after functional restoration: III. Isokinetic and isoinertial lifting capacity. Spine. 1994;18:401-415.
80 Kohles S, Barnes D, Gatchel R, et al. Improved physical performance outcomes following functional restoration treatment in patient with chronic low back pain: Early versus recent training results. Spine. 1990;15:1321-1324.
81 Rissanen A, Kalimo H, Alaranta H. Effect of intensive training on the isokinetic strength and structure of lumbar muscles in patients with chronic low back pain. Spine. 1995;20:333-340.
82 Andersson E, Sward L, Torstensson A. Trunk muscle strength in athletes. Med Sci Sports Exerc. 1988;20:587-593.
83 Mayer T, Gatchel R, Kishino N, et al. Objective assessment of spine function following industrial injury: A prospective study with comparison group and one-year follow-up. 1985 Volvo Award in Clinical Sciences. Spine. 1985;10:482-493.
84 Fricton JR, Auvinen MD, Dykstra D, et al. Myofascial pain syndrome: Electromyographic changes associated with local twitch response. Arch Phys Med Rehabil. 1985;66:314-317.
85 Gerwin RD, Shannon S, Hong CZ, et al. Interrater reliability in myofascial trigger point examination. Pain. 1997;69:65-73.
86 Hsieh CY, Hong CZ, Adams AH, et al. Inter-examiner reliability in the palpation of trigger points in the trunk and lower limb muscles. Arch Phys Med Rehabil. 2000;81:1257-1258.
87 Nice DA, Riddle DL, Lamb RL, et al. Intertester reliability of judgments of the presence of trigger points in patients with low back pain. Arch Phys Med Rehabil. 1992;73:893-898.
88 Simons DG, Travell JG. Myofascial origins of low back pain. Postgrad Med. 1983;73:65-70. 81-92, 99-105, 108
89 Cummings TM, White AR. Needling therapies in the management of myofascial trigger point pain: A systematic review. Arch Phys Med Rehabil. 2000;82:986-992.
90 Han SC, Harrison P. Myofascial pain syndrome and trigger-point management. Reg Anesth. 1997;22:89-101.
91 Irnich D, Behrens N, et al. Immediate effects of dry needling and acupuncture at distant points in chronic neck pain: Results of a randomized, double-blinded, sham-controlled crossover trial. Pain. 2002;99:83-89.
92 Simpson LL. Ammonium chloride and methylamine hydrochloride antagonize clostridial neurotoxins. J Pharmacol Exp Ther. 1983;225:546-552.
93 Simpson LL, Coffield JA, Bakry N. Chelation of zinc antagonizes the neuromuscular blocking properties of the seven serotypes of botulinum neurotoxin as well as tetanus toxin. J Pharmacol Exp Ther. 1993;267:720-727.
94 Simpson LL, Coffield JA, Bakry N. Inhibition of vacuolar adenosine triphosphate antagonizes the effects of clostridial neurotoxins but not phospholipase A2 neurotoxins. J Pharmacol Exp Ther. 1994;269:256-262.
95 Simpson LL. Kinetic studies on the interaction between botulinum toxin type A and the cholinergic neuromuscular junction. J Pharmacol Exp Ther. 1980;212:16-21.
96 Simpson LL. The origin, structure, and pharmacological activity of botulinum toxin. Pharmacol Rev. 1981;33:155-188.
97 Kalandakanond S, Coffield JA. Cleavage of intracellular substrates of botulinum toxins A, C, and D in a mammalian target tissue. J Pharmacol Exp Ther. 2001;296:749-755.
98 Styf JR, Willen J. The effects of external compression by three different retractors on pressure in the erector spine muscles during and after posterior lumbar spine surgery in humans. Spine. 1998;23:354-358.
99 Cloward RB. The treatment of ruptured intervertebral discs by vertebral body fusion. Indications, operative technique, after care. J Neurosurg. 1953;10:154.
100 Steffee AD, Sitkowski DJ. Posterior lumbar interbody fusion and plates. Clin Orthop. 1988;227:99-102.
101 Wiltse LL, Bateman J, Hutchinson RH, et al. The paraspinal sacrospinalis-splitting approach to the lumbar spine. J Bone Joint Surg. 1968;50:919-926.
102 Wiltse LL, Spencer CW. New uses and refinements of the paraspinal approach to the lumbar spine. Spine. 1988;13:696-706.
103 Carpenter N. Spondylolisthesis. Br J Surg. 1932;19:374-386.