CHAPTER 3 Local anesthetics
The peripheral nerve
Applied anatomy
The typical nerve cell has been traditionally described in terms of having a cell body (perikaryon), multiple dendrites, and a single axon (Fig. 3.1). Sensory neurons are classified as unipolar; that is, they have an axon that divides to extend a branch to both the spinal cord and the periphery. Motor neurons are classified as multipolar because, in addition to an axon, they possess many dendrites. Impulses arriving via the dendrites and cell body are integrated at the axon hillock, a specialized area of the cell body. Summation of excitatory and inhibitory impulses occurs at the axon hillock and determines whether impulses are generated or not.
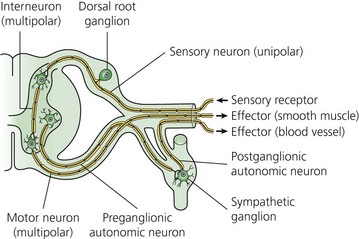
(From Ref. 1, Strichatz GR. Neural Physiology and Local Anesthetic Action. In: Cousins MJ, Bridenbaugh PO (eds). Neural blockade in clinical anesthesia and management of pain, 3rd edn. Philadelphia: © Lippincott-Raven; 1998.)
The axon is always enclosed within a nutriprotective Schwann cell envelope. Most are further invested in a myelin sheath formed by a single Schwann cell wrapped many times around the axon and interrupted periodically at the nodes of Ranvier. Many unmyelinated nerves, on the other hand, may have their axons enclosed within the folds of a single Schwann cell (Fig. 3.2).
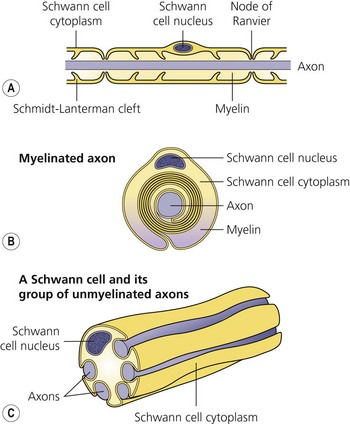
(From Ref. 1, Strichatz GR. Neural physiology and local anesthetic action. In: Cousins MJ, Bridenbaugh PO (eds). Neural blockade in clinical anesthesia and management of pain, 3rd edn. Philadelphia: © Lippincott-Raven; 1998.)
The nerve cell membrane, in common with all cells of the body, comprises a phospholipid bilayer traversed by proteins that selectively regulate the influx and efflux of ions and molecules, act as hormone and transmitter receptors, are involved in cell-to-cell interactions, and enhance the structural integrity of the membrane (Fig. 3.3). It is the specialized nature of some of these proteins that is responsible for the unique character of nerve cells.2
Ionic basis of conduction
A special membrane protein, the Na+–K+ ATPase pump, is responsible for the transmembrane concentration gradient of these ions peculiar to nerve cells. It transports sodium out of the cell and potassium into it.3 At rest, the membranse is selectively permeable to K+, resulting in an efflux of positive charge. Thus, the interior of the cell is negatively charged relative to the exterior; this resting membrane potential is in the order of 70–80 mV. Because of its chemical and electrical gradient, there is a tendency for Na+ to enter the cell.
Temporal and spatial summation of excitatory and inhibitory potentials occurs at the axon hillock. Small net depolarizations of 15–20 mV will raise the membrane potential to −55 mV, resulting in a voltage-dependent opening of Na+ channels and a rapid change in transmembrane potential to +40 mV.4–6 This is shortly followed by the opening of K+ channels, and the subsequent outward flow of K+ returns the membrane potential to normal and beyond (the refractory period where it is more difficult to stimulate the nerve).3 The Na+−K+ pump then serves to restore the chemical gradient to its initial state. These changes in transmembrane potential account for the familiar action potential (Fig. 3.4). The electrical changes occurring during the action potential serve to open adjacent voltage-dependent Na+ channels, and so the action potential is propagated along the axon. Because the area immediately preceding the action potential is in the refractory period, the action potential is propagated in one direction only.
Structure and function of local anesthetics
Local anesthetics consist of a lipophilic aromatic ring connected by a hydrocarbon chain to a hydrophilic tertiary amine (Fig. 3.5). The lipophilic moiety is responsible for the anesthetic activity of the molecule. The drugs are classified as amide or ester local anesthetics based on the nature of the bond linking the hydrocarbon chain and the aromatic ring. The ester drugs are rapidly hydrolyzed by plasma and other esterases,8–12 and have been associated with allergic and hypersensitivity reactions linked to their breakdown product para-aminobenzoic acid.13 In contrast, amides are relatively stable compounds, are metabolized in the liver, and allergic reactions to them are exceedingly rare. The comparative pharmacology of local anesthetics is shown in Table 3.1.
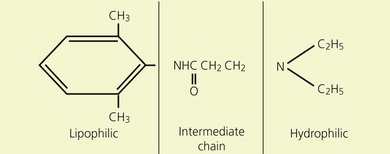
(From Ref. 7, Stoelting RK. Pharmacology and physiology in anesthetic practice. 2nd edn. Philadelphia: © JB Lippincott; 1991.)
Local anesthetics produce conduction blockade through reversible inhibition of Na+ channel function.15,16 Physiological studies have demonstrated that local anesthetics inhibit stimulated channels more readily than resting channels; this is known as phasic block and tonic block, respectively.17 The modulated receptor hypothesis has been proposed to explain these features.18,19 It is based on the fact that Na+ channels pass through various states during membrane depolarization. They begin in the resting state (R), pass through an intermediate closed form (C), to reach an open form (O), and then close to reach an inactivated state (I). According to the modulated receptor hypothesis, local anesthetics have greater affinity for Na+ channels in the O and I configurations than in the C and R configurations. Thus, local anesthetics will more readily bind Na+ channels of stimulated or active nerves.
Two possible binding sites for local anesthetics have been identified on the Na+ channel.15,18 The first site is thought to be responsible for phasic block and is situated near the channel pore. Binding and unbinding from this site is relatively slow. The second site is on the inner aspect of the channel in the hydrophobic center of the membrane. Binding and dissociation at this site is rapid.
Pharmacodynamics
Their ratio is given by the Henderson–Hasselbach equation:
Both the ionized and non-ionized forms can inhibit Na+ channels.20–23 The observations that tertiary amine local anesthetics are more potent when applied externally at an alkaline pH, or applied directly internally, suggest that the neutral form of the local anesthetic traverses the membrane, where it assumes its ionized form once again to become active at the internal aspect of the Na+ channel.24 Following injection, the alkaline pH of the tissues releases the base:
Physiochemical properties of local anesthetics
Ionization
The degree of ionization depends on the pKa of the agent and the ambient pH. The pKa is defined as the negative logarithm of the dissociation constant (Ka) of the conjugate acid. It is equal to the pH at which the local anesthetic is 50% ionized. The greater the pKa of the base, the smaller the proportion existing in its non-ionized form at any pH, and so the slower the speed of onset.25,26
Lipid solubility
The lipid solubility of local anesthetics may be expressed in terms of their water:oil partition coefficient. A high coefficient indicates a high degree of lipid solubility and ready penetration of nerve fibers. While balanced by the high fraction of drug that is therefore in the non-ionized state, in general, high lipid solubility is associated with increased potency and duration of effect.26,27
Protein binding
The duration of action of local anesthetics is related to their degree of protein binding. The bound fraction constitutes a functional reservoir that is released as the free drug is distributed or eliminated. Because it is only the unbound fraction of drug that is active, a high degree of protein binding will also result in a slower onset rate.28
Pharmacokinetics
Local distribution
where dQ/dT is the rate of passive diffusion; D the diffusion coefficient of the drug in the membrane; A the area of the membrane; K the aqueous membrane partition coefficient of the drug; ΔC the concentration gradient; and δ the thickness of the membrane.
Local clearance of drug depends on the vascularity of the injection site and the degree of tissue binding. Therefore a rich capillary bed and little surrounding fatty tissue coupled with a low water:oil partition coefficient favors systemic absorption. The rate of absorption, and hence initial plasma concentrations as a function of site of injection, vary as follows: spinal < plexus block < epidural < caudal < intercostal < intrapleural (Fig. 3.6).
Following absorption into the systemic circulation, local anesthetics are subjected to substantial sequestration by the lungs.30,31 This is because of a high lung:blood partition coefficient and ion trapping of drug secondary to the low extravascular pH of the lungs. The drugs also bind plasma proteins, showing high affinity and low capacity for alpha1-acid glycoprotein, and low affinity and high capacity for albumin. This binding is increased in the presence of cancer, trauma, chronic pain, and inflammatory disease, as well as in the postoperative period; it is significantly decreased in neonates because of their low plasma concentrations of alpha1-acid glycoprotein. Further binding of drug takes place in the tissue. The long-acting group of amide local anesthetics are bound in plasma and tissue to a greater extent than the short-acting ones.32–37
The half-life of elimination can be calculated following the intravenous injection of a bolus of drug. It allows one to anticipate the risk of drug accumulation in case of reinjection. For example, lidocaine has an elimination half-life of 96min and bupivacaine 210 min.14 Therefore as a rough guide one may readminister half the initial dose 1.5 and 3.5h following the first injection, and in this way avoid drug accumulation.
Metabolism and excretion
Amide local anesthetics are metabolized in the liver and their elimination depends on their hepatic clearance. They can be divided into two groups, depending on whether their hepatic extraction ratio is high (e.g. lidocaine, >50%) or low (e.g. bupivacaine, <40%). Those drugs with a high ratio have, therefore, perfusion-dependent clearance; those with a low ratio are subject to induction and inhibition of hepatic enzyme systems.38
As stated above, the ester drugs are rapidly hydrolyzed by plasma and other esterases, limiting their potential for toxicity.8,9,11,39,40 Renal excretion of local anesthetics is of little importance, accounting for less than 6% of the dose. This may be increased, however, to 20% following acidification of the urine.41
Nerve block in clinical practice
Nerve fibers
Nerve fibers have been categorized into A, B, and C fibers. A fibers have been further divided into α, β, γ, and δ fibers. The important features of each category of nerve fiber are outlined in Table 3.2. A fibers are myelinated somatic nerves, B fibers are myelinated preganglionic autonomic nerves, and C fibers are unmyelinated nerves. The susceptibility of nerves to local anesthetics, in general, depends on their caliber, degree of myelination, and speed of conduction. However, as outlined below, further factors also come into play.
Minimum blocking concentration
The minimum blocking concentration (Cm) is the lowest concentration of a local anesthetic agent that will block conduction in a nerve in vitro. In vivo, the drug is injected in and about nerve trunks, fibrous sheaths, fatty tissue, and blood vessels. Therefore, before reaching a nerve, it is subject to dilution, dispersion, fixation, destruction, and systemic absorption. Under these conditions, the minimum concentration necessary to block a nerve is much greater than the Cm. Consequently, lidocaine 1% is necessary to block a mixed somatic nerve that has a Cm for lidocaine of approximately 0.07%.42
Differential nerve block
Within a single peripheral nerve, one may observe complete block of pain fibers (Aδ and C) while motor and touch (Aα and Aβ) are spared. This is known as differential nerve block. A number of possible explanations for this phenomenon have been postulated. First, the time taken for a drug to diffuse into and along the course of a nerve, and so affect various fibers, may result in the clinical features observed. Second, the presence or absence of a myelin sheath may affect local anesthetic activity and penetration. Third, not all axons have the same sensitivity to local anesthetic agents because of variations in Na+ channel and membrane lipid content.43,44
Nerve penetration
Peripheral nerves are organized so that the fibers innervating the distal portions of a limb are in the center of the nerve trunk and the more proximal structures are supplied from the outer layers of the trunk. Following deposition of the drug, one may therefore observe anesthesia of the more proximal limb structures before the distal ones (Fig. 3.7).
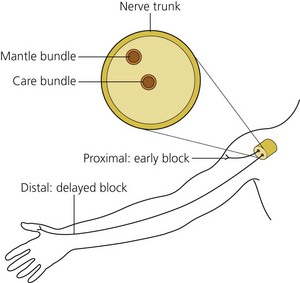
(From Ref. 45, de Jong RH. Physiology and pharmacology of local anesthesia. Springfield, IL, 1970. Courtesy of Charles C. Thomas Publishers, Ltd, Springfield, Illinois, USA.)
Regression of block is primarily dependent on diffusion from the nerve and absorption into the local vasculature. Drugs with high lipophilic solubility diffuse slowly from local tissues for reasons stated earlier, while the addition of adrenaline to local anesthetics results in local vasoconstriction and an increase of up to 50% in block duration.46–48
1 Strichartz GR. Neural physiology and local anesthetic action. In: Cousins MJ, Bridenbaugh PO, editors. Neural blockade in clinical anesthesia and management of pain. 3rd edn. Philadelphia: Lippincott-Raven; 1998:35-54.
2 Kandel ER, Schwartz JH, Jessel T, editors. Principles of neural science, 2nd edn, New York: Elsevier/North-Holland, 1992.
3 Rang HP, Ritchie JM. On the electrogenic sodium pump in mammalian non-myelinated nerve fibers and its activation by various cations. J Physiol. 1968;196:183-221.
4 Hille B. Ionic channels of excitable membranes, 2nd edn. Sunderland, MA: Sinauer Associates; 1991.
5 Hodgkin AL, Huxley AF. A quantitative description of membrane current and its application to conduction and excitation in nerve. J Physiol. 1952;117:500-544.
6 Stühmer W, Conti F, Harukazu S, et al. Structural parts involved in activation and inactivation of the sodium channel. Nature. 1989;339:565-644.
7 Stoelting RK. Pharmacology and physiology in anesthetic practice, 2nd edn. Philadelphia: JB Lippincott; 1991.
8 Kuhnert PM, Kuhnert BR, Philipson EH, et al. The half-life of 2-chloroprocaine. Anesth Analg. 1986;65:273-278.
9 O’Brien JE, Abbey V, Hinsvark O, et al. Metabolism and measurement of chloroprocaine, an ester-type local anesthetic. J Pharm Sci. 1979;68:75-78.
10 DuSouich P, Erill S. Altered metabolism of procainamide and procaine in patients with pulmonary and cardiac diseases. Clin Pharmacol Ther. 1977;21:101.
11 Reidenberg MM, James M, Dring LG. The rate of procaine hydrolysis in serum of normal subjects and diseased patients. Clin Pharmacol Ther. 1972;13:279-284.
12 Foldes FF, Davidson GN, Duncalf D, et al. The intravenous toxicity of local anesthetic agents in man. Clin Pharmacol Ther. 1965;40:328-335.
13 Fisher MM, Graham R. Adverse responses to local anaesthetics. Anaesth Intensive Care. 1984;12:325-327.
14 Covino BG, Vassalo HL. Local anesthetics: mechanisms of action and clinical use. New York: Grune and Stratton; 1976. 73
15 Butterworth JF, Strichartz GR. Molecular mechanisms of local anesthesia: a review. Anesthesiology. 1990;72:711-734.
16 Cahalan M, Shapiro BI, Almers W. Relationship between inactivation of sodium channels and block by quarternary derivatives of local anesthetics and other compounds. In: Fink BR, editor. Molecular mechanisms of anesthesia (Progress in anesthesiology, Vol. 2). New York: Raven Press, 1980.
17 Courtney KR. Structure-activity relations for frequency-dependent sodium channel block in nerve by local anesthetics. J Pharmacol Exp Ther. 1980;213:114-119.
18 Hille B. Local anesthetics: hydrophilic and hydrophobic pathways for the drug-receptor reaction. J Gen Physiol. 1977;69:497-515.
19 Hille B. Local anesthetic action on inactivation of the Na+ channel in nerve and skeletal muscle: possible mechanisms for antiarrhythmic agents. In: Morad M, editor. Biophysical aspects of cardiac muscle. New York: Academic Press; 1978:55-74.
20 Frazier DT, Narahashi T, Yamada M. The site of action and active form of local anesthetics. II. Experiments with quaternary compounds. J Pharmacol Exp Ther. 1970;171:45-51.
21 Strichartz GR. The inhibition of sodium currents in myelinated nerve by quaternary derivatives of lidocaine. J Gen Physiol. 1973;62:37-57.
22 Chernoff DM, Strichartz GR. Tonic and phasic block of neuronal sodium currents by 5-hydroxyhexano-2′,6′-xylidide, a neutral lidocaine homologue. J Gen Physiol. 1989;93:1075-1090.
23 Ritchie JM, Ritchie BR. Local anaesthetics: effect of pH on activity. Science. 1968;162:1394-1395.
24 Narahashi T, Frazier D, Yamada M. The site of action and active form of local anesthetics. I. Theory and pH experiments with tertiary compounds. J Pharmacol Exp Ther. 1970;171:32-44.
25 Sanchez V, Arthur GR, Strichartz G. Fundamental properties of local anesthetics. I. The dependence of lidocaine’s ionization and octanol:buffer partitioning on solvent and temperature. Anesth Analg. 1987;66:159-165.
26 Strichartz GR, Sanchez V, Arthur GR, et al. Fundamental properties of local anesthetics. II. Measured octanol:buffer partition coefficients and pKa values of clinically used drugs. Anesth Analg. 1990;71:158-170.
27 Truant AP, Takman B. Differential physical-chemical and neuropharmacologic properties of local anesthetic agents. Anesth Analg. 1959;38:478-484.
28 Tucker GT. Plasma binding and disposition of local anesthetics. Int Anesthesiol Clin. 1975;13:33-59.
29 Tucker GT, Moore DC, Bridenbaugh PO, et al. Systemic absorption of mepivacaine in commonly used regional block procedures. Anesthesiology. 1972;37:277-287.
30 Jorfeldt L, Lewis DH, Lofstrom B, et al. Lung uptake of lidocaine in healthy volunteers. Acta Anaesthesiol Scand. 1979;23:567-574.
31 Lofstrom B. Tissue distribution of local anesthetics with special reference to the lung. Int Anesthesiol Clin. 1978;16:53-71.
32 Denson DD, Coyle DE, Thompson G, et al. Alpha1-acid glycoprotein and albumin in human serum bupivacaine binding. Clin Pharmacol Ther. 1984;35:409-415.
33 Kraus E, Polnaszek CF, Scheeler DA, et al. Interaction between human serum albumin and alpha1-acid glycoprotein in the binding of lidocaine to purified protein fractions and sera. J Pharmacol Exp Ther. 1986;239:754-759.
34 Mather LE, Long GJ, Thomas J. The binding of bupivacaine to maternal and foetal plasma proteins. J Pharm Pharmacol. 1971;23:359-365.
35 Mather LE, Thomas J. Bupivacaine binding to plasma protein fractions. J Pharm Pharmacol. 1978;30:653-654.
36 Routledge PA, Barchowsky A, Bjornsson TD, et al. Lidocaine plasma protein binding. Clin Pharmacol Ther. 1980;27:347-351.
37 Tucker GT, Boyes RN, Bridenbaugh PO, et al. Binding of anilide-type local anesthetics in human plasma. I. Relationships between binding, physiochemical properties and anesthetic activity. Anesthesiology. 1970;33:287-303.
38 Tucker GT. Pharmacokinetics of local anaesthetics. Br J Anaesth. 1986;58:717-731.
39 Calvo R, Carlos R, Erill S. Effects of disease and acetazolamine on procaine hydrolysis by red cell enzymes. Clin Pharmacol Ther. 1980;27:179-183.
40 Javaid JI, Musa MN, Fischman M, et al. Kinetics of cocaine in humans after intravenous and intranasal administration. Biopharm Drug Dispos. 1983;4:9-18.
41 Tucker GT, Mather LE. Clinical pharmacokinetics of local anaesthetic agents. Clin Pharmacokinet. 1979;4:241-278.
42 Gissen AJ, Covino BG, Gregus J. Differential sensitivity of mammalian nerve fibers to local anesthetic drugs. Anesthesiology. 1980;53:467-474.
43 Heinbecker P, Bishop GH, O’Leary J. Pain and touch fibers in peripheral nerves. Arch Neurol Psychiatr. 1933;20:771-789.
44 Raymond SA, Gissen AJ. Mechanisms of differential block. In: Strichartz GR, editor. Handbook of experimental pharmacology, Vol. 81. Berlin: Springer-Verlag; 1987.
45 de Jong RH. Physiology and pharmacology of local anesthesia. Springfield, IL: Charles C Thomas; 1970.
46 Kristerson L, Nordenram Å, Nordqvist P. Penetration of radioactive local anaesthetic into peripheral nerve. Arch Int Pharmacodyn. 1965;157:148-151.
47 Winnie AP, LaVallee DA, Sosa BP, et al. Clinical pharmacokinetics of local anesthetics. Can Anaesth Soc J. 1977;24:252.
48 Winnie AP, Tay CH, Patel KP, et al. Pharmacokinetics of local anesthetics during plexus blocks. Anesth Analg. 1977;56:852-861.