CHAPTER 56 Laryngeal and Pharyngeal Function
The upper aerodigestive tract serves the competing functions of respiration and swallowing. The nose is the primary respiratory orifice, and the mouth is the portal for ingestion of food. Both open into a common cavity, the pharynx. The patency of the upper airway must be actively supported during breathing, yet total and forceful collapse is required to propel food into the esophagus during swallowing. The airway must be protected during a swallow so that ingested food or water does not spill into the trachea. Aspiration of food or foreign material can lead to serious consequences, such as asphyxia or lung infection. In humans, the function of the upper aerodigestive is considerably more complex, owing to the demands of speech as well as to a significant structural difference. In infants and in all nonhuman mammals, the pharynx is functionally compartmentalized into separate passages for breathing and alimentation. The epiglottis interdigitates with the uvula to form a respiratory channel from the nose into the larynx and two lateral pathways from the mouth to the esophagus through the pyriform sinuses.1 During postnatal development in humans, enlargement of the cranium with flexion of the base of the skull results in a downward displacement of the larynx. This displacement elongates the pharynx and distracts the uvula and epiglottis so that they are no longer in contact. The result is a common pharyngeal cavity for breathing and swallowing (Fig. 56-1).2,3 The larynx begins its descent at the age of about 18 to 24 months. There are two positive outcomes. Vocal power is greater because of increased resonance, and articulatory diversity is expanded.4
This complicated and potentially hazardous configuration of the upper airway results from embryology and reflects evolution. The lower respiratory tract has evolved as an offshoot of the digestive tract, first appearing in the lungfish as a simple muscle sphincter to protect the lungs from water.5 Consequently, during embryologic development, the foregut is the common origin of the larynx, trachea, and esophagus.
Laryngeal Motion
Applied Anatomy
In the illustrations of many textbooks, the membranous vocal folds are depicted as moving solely in the axial plane, with rotational motion similar to that of a windshield washer. Details of motion of the posterior, cartilaginous portions of the larynx have been largely ignored. The reason is that early concepts of motion were based on observing laryngeal motion with a mirror, and then recording the observations with two-dimensional freehand drawings. However, with the advent of flexible endoscopy, stroboscopy, videorecording, and computerized imaging, it has become clear that laryngeal motion is more complex than previously recognized. Vocal folds move in three dimensions and undergo conformational changes in length, shape, and volume (Fig. 56-2).6 The terms cadaveric and paramedian have been commonly used to describe the position of paralyzed vocal folds. These terms are inadequate to describe the three-dimensional changes in configuration of the glottis in paralysis.7 Motion of the larynx is best understood as the net result of the interaction of its component parts.
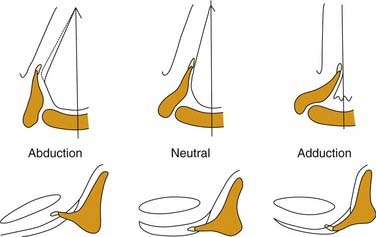
Figure 56-2. Three-dimensional motion of the arytenoid cartilage and vocal fold.
(From Hirano M. Anatomy and behavior of the vocal process. In: Baer T, Sasaki C, Harris K, eds. Laryngeal Function in Phonation and Respiration. Boston: College-Hill Press; 1987.)
The laryngeal skeleton consists of the hyoid bone and a series of cartilages. The hyoid bone is a U-shaped structure, open posteriorly, which is suspended from the base of the skull and mandible by muscles and ligaments. The thyroid cartilage, the largest cartilage in the larynx, is suspended from the hyoid bone. The word thyroid means “shield” and this name is appropriate, because the structure is not only shaped like a shield but also provides support and protection for the vocal folds. In the axial plane, the thyroid cartilage is shaped like a V, with two wings that project posteriorly. Like the hyoid bone, the thyroid cartilage is open posteriorly. The vocal folds attach to the anterior inner surface of the thyroid cartilage. The posterior ends of the vocal folds are anchored to the arytenoid cartilages, which are the chief moving parts of the larynx. The arytenoid cartilages sit atop the posterior rim of the cricoid cartilage and articulate via shallow ball-and-socket joints. The cricoid cartilage is the only complete rigid ring within the airway. It is shaped like a signet ring and is broadest posteriorly. Inferiorly, and just lateral to the cricoid joints, the inferior cornua of the thyroid cartilage articulates with the cricoid in two hingelike joints, creating a visor-like, or “bucket handle” structure, with motion that controls the space between the anterior rims of the thyroid and cricoid cartilages.8
Most intrinsic laryngeal muscles connect the arytenoid cartilages to either the cricoid or thyroid cartilages. These include the thyroarytenoid muscle, the lateral cricothyroid muscle, the interarytenoid muscle, which act to adduct the vocal folds, and the posterior cricoarytenoid muscle, which is the only active abductor of the larynx. As mentioned above, the arytenoid cartilages are the chief moving parts of the larynx. The membranous vocal folds are suspended between the thyroid cartilage and the arytenoids, and the position of each membranous vocal fold is determined by the motion of the arytenoid cartilage. The arytenoid rotates upward and outward to open the larynx, and rotates inward to close it. Contraction of the posterior cricoarytenoid muscle pulls the muscular process of the arytenoid posteriorly and caudally. The structure of the cricoarytenoid joint prevents the entire arytenoid from being pulled along this vector. Instead, the arytenoid rotates, displacing the vocal process upward and laterally, abducting the vocal fold (Fig. 56-3).9 Conversely, the lateral cricoarytenoid muscle pulls the muscular process of the arytenoid anteriorly and caudally, which rotates the arytenoid, moving the vocal process medially and adducting the vocal fold. The interarytenoid muscles pull the arytenoid cartilages together. The thyroarytenoid muscles exert some adductor force, but their major action is to shorten or tense the vocal fold and increase its cross-sectional area (Fig. 56-4).
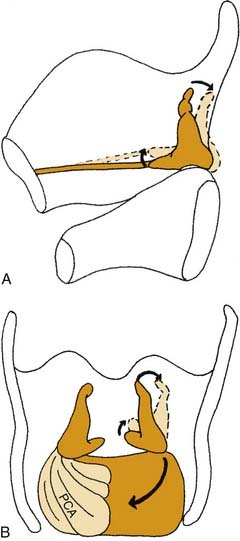
Figure 56-3. Three-dimensional effects of posterior cricoarytenoid muscle contraction. A, Sagittal view. B, Posterior view.
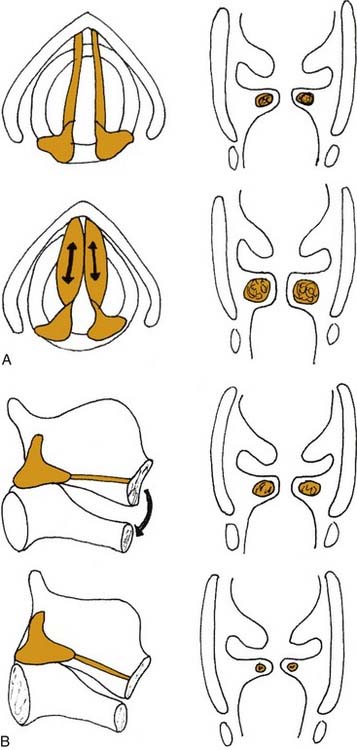
Figure 56-4. Effects of (A) thyroarytenoid and (B) cricothyroid muscle contraction on the thickness of the vocal fold.
In addition to the diverse force vectors of individual laryngeal muscles, there is also segmental compartmentalization within muscles, increasing the possibilities for fine control. For example, the human posterior cricoarytenoid muscle is divided into two compartments. These are supplied by separate nerve branches, differ in fiber type, and insert on opposing sides of the muscular process (Fig. 56-5).9,10,11 The human thyroarytenoid muscle is also compartmentalized: It has long been regarded to have a separate medial compartment, the “vocalis.”
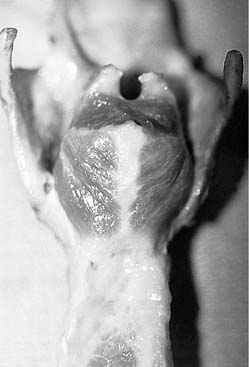
Figure 56-5. Compartmentalization of the human posterior cricoarytenoid muscle. Posterior view of a cadaver larynx.
In contrast to other intrinsic laryngeal muscles, the cricothyroid muscle does not insert on the arytenoid cartilage and therefore has no direct action on arytenoid motion. Instead, the cricothyroid muscle connects the anterior edges of the thyroid and cricoid cartilages. Contraction of this muscle pulls the two cartilages closer together, which increases the distance between the anterior commissure and the cricoid. The result is stretching of the vocal fold, increasing its length and tension. Because both vocal folds insert on the anterior commissure, contraction of either cricothyroid muscle affects both ipsilateral and contralateral vocal folds. Contraction of both right and left cricothyroid muscles results in maximal anterior traction.8
Muscles that are extrinsic to, but connect with, the larynx can also affect glottic function by exerting traction on the laryngeal cartilages. The sternohyoid, thyrohyoid, and omohyoid muscles, innervated by the ansa cervicalis, exert caudal traction on the larynx. This action, as well as downward traction on the trachea during inspiration, causes abduction of the vocal folds.12,13 Muscles that exert a cephalad force include the geniohyoid, anterior belly of the digastric, mylohyoid, and stylohyoid muscles. In patients with hyperfunctional dysphonia, excess activity can usually be palpated in the extrinsic laryngeal muscles.
Laryngeal Function in Breathing
The primary and most primitive function of the larynx is to protect the lower airway. In evolution, the larynx first appeared as a sphincter to prevent the ingress of water into the airway of the lungfish.5 Subsequently, dilator muscles evolved to permit active opening of the larynx. In more evolved animals, the larynx is not just an open or shut valve but, rather, a variable resistor, capable of regulating airflow. Other laryngeal functions are the Valsalva maneuver and coughing. The larynx is also a sensory organ, providing information about airway function and the purity of inhaled air and serving in the afferent limb of many reflexes.
Protection
When the larynx is mechanically stimulated, it closes abruptly, and respiration ceases. Apnea can also occur in response to such diverse chemical agents as ammonia, phenyl diguanide, and cigarette smoke. These are appropriate and beneficial responses that prevent the entry of foreign matter into the lower airway, although strong laryngeal stimulation may result in responses that appear to be maladaptive, such as laryngospasm and prolonged bronchoconstriction.14 These reflexes may be produced in experimental animals by electrical stimulation of the superior laryngeal nerve and probably represent an oversaturation of pathways that serve a useful function at lower levels of input.
Recurrent paroxysmal laryngospasm is occasionally encountered in clinical practice. In some patients, it is caused by gastroesophageal reflux, which responds to acid-suppressing medication. In other patients, the pathophysiology appears to be a hypersensitive laryngeal closure reflex, because such patients report some triggering event such as eating or inhaling steam or odors. The onset frequently occurs during an upper respiratory infection, but it also can occur after surgical trauma to the recurrent laryngeal nerve. Most often the condition resolves spontaneously within a few months, but it may become a permanent and debilitating problem. The laryngeal closure reflex is particularly sensitive in infants and can be elicited by a stimulus as weak as water. During early infancy, the strength of this reflex increases, then decreases, along a time course similar to that of the incidence of sudden infant death syndrome, suggesting that laryngeal reflexes may play a role in its cause.15
Cough
Another important protective reflex involving the larynx is the cough, which ejects mucus and foreign material from the lungs.16 Cough can be a voluntary action or a reflexive response to stimulation of the larynx or receptors in the lungs. The cough reflex is suppressed during sleep, so that a greater stimulus is required with progressive stages of sleep. During deep sleep, a cough cannot be elicited unless the stimulus first results in arousal to a lighter level of sleep.
Control of Ventilation
The role of the larynx as an active organ of respiration is not widely recognized. Abduction and adduction of the larynx in phase with respiration has been acknowledged for many years.17,18 It has been postulated that all respiratory motion of the larynx is passive, resulting from biomechanical coupling of the larynx to the tracheobronchial tree.12,19 There is some evidence that downward traction on the larynx dilates the glottis.13 But clinical observations and experimental evidence indicate that the larynx moves actively in respiration and, in fact, plays an important role regulating respiration. The larynx is located at the entrance into the trachea and is capable not only of rapid opening and closure but also of sudden alterations in resistance. Hence, the larynx is better adapted than any other portion of the respiratory tract for regulating airflow. The resistance changes resulting from laryngeal responses to respiratory stimuli, such as negative airway pressure and blood gas changes, have a beneficial effect on ventilation.20
Widening of the glottis during inspiration is a primary action of ventilation that ceases only during deep anesthesia or sleep. The posterior cricoarytenoid muscle (PCA), the only active dilator of the larynx, begins to contract with each inspiration before activation of the diaphragm.5,21,22
The level of PCA activity, and hence laryngeal movement with breathing, varies. Laryngeal motion may be imperceptible during unlabored, quiet breathing. With increasing respiratory drive, peak inspiratory PCA activity increases proportionately with diaphragmatic activity. There are important differences between PCA activity and diaphragmatic behavior (Fig. 56-6). When the upper airway is partially occluded, inspiration generates negative airway pressure, which is a potent stimulus to the PCA and the several other upper airway muscles that dilate the upper airway.20,23 In contrast, the diaphragm responds by actually decreasing inspiratory force and by increasing the duration of inspiration.14 This response occurs because, during partial airway obstruction, the PCA and the diaphragm have opposing effects on patency of the lumen. Increasing diaphragmatic force raises the negative pressure, favoring airway collapse. To inspire the same volume, the diaphragm extends the duration of inspiration. PCA contraction dilates the airway, opposing the effects of the diaphragm. During strong respiratory demand, the PCA continues contracting during expiration after the diaphragm has relaxed, thus delaying expiratory adduction and facilitating the outflow of air. During panting, the glottis sustains an abducted posture, ensuring maximal airflow. Because of these physiologic differences, the phrenic nerve is not an ideal choice for reinnervation of the PCA in patients with laryngeal paralysis.
There has been some controversy in the literature as to whether laryngeal adduction during expiration is an active or passive process. Some electromyographic studies have not confirmed any expiratory activity of laryngeal adductor muscles, suggesting that expiratory narrowing of the glottis is passive, a result of relaxation of the PCA.24,25 Other researchers have documented phasic expiratory activity of laryngeal adductors.26,27 These conflicting observations have been reconciled by the finding that respiratory activity of laryngeal adductor muscles is much less consistent than that of the PCA. Expiratory adduction of the larynx is either active or passive, depending on breathing strategy. During baseline conditions, such as sleep, laryngeal adductor muscles are silent. During consciousness, expiratory activation of laryngeal adductor muscles is frequently detected, but at highly variable levels. Correlations of laryngeal adductor muscle activity with expiratory airflow, ventilatory pattern, and transglottal resistance suggest that this is an important mechanism for controlling the rate of breathing (Fig. 56-7).28 Glottic adduction increases expiratory resistance to airflow, prolonging exhalation. During normal breathing conditions, respiratory rate is primarily controlled by variation in the rate of exhalation.29 Exhalation is slowed by partial contraction of the diaphragm during expiration. Abdominal muscle pushing increases the rate of exhalation, although during tidal breathing under baseline conditions, the larynx appears to be the primary mechanism for controlling the ventilatory pattern.
Sensory Receptors
The larynx is not only a motor organ. It also is the location of a variety of sensory receptors that exert influences on breathing and cardiovascular function. The larynx is densely supplied with sensory receptors; sensory fibers from the larynx are several times more numerous than those from the lungs. This finding is remarkable when one considers that the internal surface area of the lungs is several square meters, whereas the larynx is a small orifice. Single nerve fiber recordings from the superior laryngeal nerve have identified three major types of laryngeal respiratory receptors: negative pressure, airflow, and “drive.”30 These receptors are activated by the process of breathing and influence the central control of breathing. Airflow receptors actually respond to a decrease in temperature because the larynx is cooled by inspired air. Thus, flow receptors do not respond to air that has been warmed and humidified by the nose. They are activated by air that comes in through the mouth, particularly in cold and dry weather. “Drive” receptors are probably proprioceptors that respond to the respiratory motion of the larynx. Laryngeal sensations of touch and chemical stimuli are not activated during normal breathing conditions, but stimulation can profoundly affect ventilation.
Circulatory Reflexes
Stimulation of the larynx can alter heart rate and blood pressure. During induction of general anesthesia, endotracheal intubation can result in bradycardia. The direct result of experimental laryngeal stimulation on blood pressure is hypertension,31 although in the clinical situation, the effects of bradycardia or ectopy usually prevail, resulting in hypotension. In patients with obstructive sleep apnea, negative airway pressure may stimulate receptors in the larynx so strongly that cardiac arrhythmias occur. Animal experiments show that the afferent limb is the superior laryngeal nerve because transection of this nerve abolishes cardiovascular responses (Fig. 56-8). The efferent limb for bradycardia is the vagus nerve, and the efferent limb for blood pressure elevation is via sympathetic nerve fibers, but intervening central connections have not been identified.32
Pharyngeal Function in Breathing
The upper airway is a conduit with several points wherein the shape and cross-sectional area can be dynamically altered. The pharynx is the largest but most compliant region, and it is susceptible to passive collapse. Maintenance of patency requires the action of striated upper airway muscles in coordination with the activity of respiratory pump muscles.33 Upper airway muscles also determine whether air is inspired through the nose or the mouth. The anatomy and intrinsic properties of upper airway muscles indicate that they are primarily adapted for nonrespiratory functions but can be used in respiratory tasks. Most of the muscles acting on the pharynx show no respiratory activity during awake, tidal breathing, but they are recruited by increased respiratory drive or upper airway obstruction (Fig. 56-9).34
In healthy patients, the nose is the preferred route of breathing, because the relaxed position of the mandible closes the mouth and the relaxed palate occludes the oropharyngeal inlet. Furthermore, the nose warms, humidifies, and filters inspired air. The selection of oral or nasal breathing is performed primarily by the soft palate, a band of moveable soft tissue suspended from the posterior bony palate. Oral breathing requires activation of the levator veli palatine to elevate the soft palate as well as activation of the musculus uvula. Nasal breathing is favored by constriction of the oropharyngeal passage, which is accomplished primarily through activation of the palatoglossal muscles, medialization of the faucial arches, and elevation of the base of tongue. This activity is highest during forced nasal breathing with the mouth open.35
Little objective information is available regarding the respiratory function of the pharyngeal constrictors. It is widely assumed that some active tone in these muscles increases the stiffness of these muscles, thereby decreasing the tendency of the pharynx to collapse with the negative pressure generated during inspiration. Conversely, flaccidity of the constrictors is considered to destabilize the airway, promoting collapse, although there are no physical data to support this concept.5 On the contrary, contraction of the constrictor muscles actively collapses the pharyngeal lumen. Spontaneous activity in the respiratory cycle has been detected in the superior constrictor muscle, but this activity occurs during the expiratory phase and disappears with bronchoconstriction, suggesting that it plays a role in modulating expiratory airflow resistance.36
The dilator muscles of the pharynx are located anteriorly and laterally. The best-studied and probably most important pharyngeal dilator is the genioglossus (GG), a fan-shaped muscle that originates from the anterior mandible and spreads out to insert into the tongue. Although no studies have conclusively established what muscles are responsible for pulling the base of the tongue forward to dilate the airway, evidence strongly supports the role of the GG. In animal experiments, increased electromyographic activity of the GG is associated with a greater capacity of the pharynx to withstand negative collapsing pressure.37 GG electromyographic activity increases reflexively in response to negative upper airway pressure (Fig. 56-10).14,23 In humans with obstructive sleep apnea, decreased GG electromyographic activity has been noted during obstructive events, whereas obstruction is relieved with recovery of GG activity.38
The hyoid bone supports the hypopharynx. In humans, as in other primates, the hyoid does not articulate with any other skeletal element; rather, it is suspended by muscles and ligaments. Contraction of muscles attached to the hyoid has been shown in animal experiments to increase the size and stability of the upper airway.39 Muscles attached to the hyoid are also believed to resist the downward traction exerted on the airway by the trachea during inspiration.35,40
Function in Speech
Phonation
The role of the larynx in sound production has been recognized for centuries,41 although the mechanism of how the larynx generates sound from exhaled air was not clear until the mid-20th century. In 1950, Husson42 presented the neurochronaxic hypothesis, which held that glottic vibrations were caused by rhythmic impulses in the nerves to the larynx, synchronous with the frequency of the sound produced, so that each vibratory cycle was caused by a separate neural impulse. This hypothesis is physiologically impossible.
In the 1950s von Leden used high-speed motion pictures to document the motion of the vocal folds during vibration and subsequently reported his theory of the mechanism of phonation; now widely accepted, the theory holds that the interaction of aerodynamic forces and the mechanical properties of the laryngeal tissues are responsible for inducing vocal fold vibration and generating vocal sound.43
Normal phonation requires that five conditions be satisfied, as listed in Box 56-1. There should be adequate breath support to provide power, and the vibratory edges of the vocal folds should be aligned and separated by an appropriately small gap. The physical properties of the vocal fold should be conducive to vibration, and its three-dimensional contour should be favorable. Finally, a normal voice requires volitional control of glottic length, tension, and shape.
Actual phonation is more complex than the previous model because the vocal fold is not a homogeneous structure and also because it vibrates in three dimensions.44 Moreover, the pattern of vibration varies with pitch and vocal register. The “body-cover” concept of phonation is that vibration of the mucosa does not correspond directly to that of the rest of the vocal fold.45 Instead, the “body” of the vocal fold is relatively static, whereas the wave is propagated in the mucosal “cover.” This mucosal wave begins on the inferomedial aspect of the vocal fold and moves rostrally (Fig. 56-11). As the superior edges of the vocal fold begin to separate, the lower edges close, and this temporal relationship is accounted for by the two-mass model proposed by Ishizaka and Flanagan.46 As the superior edges of the vocal folds separate, airflow through the divergent glottis generates greater negative pressure at the lower edge of the vocal folds, accelerating closure of the inferior glottis. The body-cover theory and the two-mass model are consistent with most of the observed motion during modal phonation (e.g., chest register, in the middle range of pitch), although the mucosal wave decreases at higher pitches and is not visible during falsetto, suggesting that the motions of the mucosa and the underlying tissue become coupled. In this mode, elastic recoil, rather than the Bernoulli effect, is the primary force driving the closing phase of phonation. The closing phase is much shorter, and only the superior edges of the vocal folds make contact. The vibratory characteristics of falsetto have been attributed to increased tension and decreased thickness of the vocal fold. During phonation at low pitches, the vocalis muscle is relaxed so that the “body” of the fold participates in oscillation.
Vibratory Capacity of the Vocal Folds
The physical properties of the vocal folds are crucial in determining vocal function. During normal modal phonation, the mucosa undulates freely over the underlying vocal ligament and vocalis muscle. Hirano’s47 important histologic studies showed that this undulation is possible because the mucosa and muscle are separated by a specialized layer of connective tissue that serves as a shock absorber. This highly specialized tissue is characterized by stratified concentrations of elastin and collagen. The most superficial layer is made up of loosely connected fibers of collagen and elastin. This layer also is known as Reinke’s space. The intermediate layer is composed predominantly of elastic fibers, and the deep layer is constructed of densely arranged collagen fibers. Together, the intermediate and deep layers form the vocal ligament.
Articulation
The source-filter hypothesis of speech states that the larynx is the source of a constant sound, which is shaped into words by the upper vocal tract. In this generally accepted model, consonants and vowels are formed by the action of the lips, tongue, palate, and pharynx. Participation of the larynx during articulation is generally considered to be limited to the onset and offset of phonation, coordinating with upper articulators to produce voiced and unvoiced sounds. In computerized simulation, this model seems to account for speech fairly well, although evidence now suggests that the position and shape of the glottis may actually vary with production of different vowels, so that the contribution of the larynx to phonation may be more complex than previously recognized.48
Laitman JT, Reidenber JS. Advances in understanding the relationship between the skull base and larynx with comments on the origins of speech. Hum Evol. 1988;3:99.
Remmers JE, deGroot WJ, Sauerland EK, et al. Pathogenesis of upper airway occlusion during sleep. J Appl Physiol. 1978;44:931.
Sant’Ambrogio G, Mathew OP, Fisher JT, et al. Laryngeal receptors responding to transmural pressure, airflow, and local muscle activity. Resp Physiol. 1983;54:317.
1. Laitman JT, Crelin ES. Developmental change in the upper respiratory system of human infants. Perinatol Neonatol. 1980;4:15.
2. Laitman JT, Crelin ES. Postnatal development of the basicranium and vocal tract region in man. In: Bosma JF, editor. Symposium on Development of the Basicranium. Washington, DC: U.S. Government Printing Office; 1976:206-220.
3. Sasaki CT, Levine PA, Laitman JT, et al. Postnatal descent of the epiglottis in man: a preliminary report. Arch Otolaryngol Head Neck Surg. 1977;103:169.
4. Laitman JT, Reidenber JS. Advances in understanding the relationship between the skull base and larynx with comments on the origins of speech. Hum Evol. 1988;3:99.
5. Negus V. The Comparative Anatomy and Physiology of the Larynx. London: Heinemann; 1949.
6. Hirano M, Yoshida T, Kurita S, et al. Anatomy and behavior of the vocal process. In: Baer T, Sasaki C, Harris K, editors. Laryngeal Function in Phonation and Respiration. Boston: College-Hill Press; 1987:3-13.
7. Woodson GE. Configuration of the glottis in laryngeal paralysis I: clinical study. Laryngoscope. 1993;103:1227.
8. Woodson GE, Murry MP, Schweizer B, et al. Unilateral cricothyroid contraction and glottic configuration. J Voice. 1998;12:335-339.
9. Bryant NJ, Woodson GE, Kaufman K, et al. Human posterior cricoarytenoid muscle compartments: anatomy and mechanics. Arch Otolaryngol. 1996;122:1331.
10. Sanders I, Wu BL, Mu L, et al. The innervation of the human larynx posterior cricoarytenoid muscle: evidence for at least two neuromuscular compartments. Laryngoscope. 1994;104:880.
11. Zemlin WR, Davis P, Gaza C. Fine morphology of the posterior cricoarytenoid muscle. Folia Phoniatr (Basel). 1984;36:233.
12. Fink RB, Demarast RJ. Laryngeal Biomechanic. Cambridge, Massachusetts: Harvard University Press; 1978.
13. Woodson GE, Sant’Ambrogio F, Mathew O, et al. Effects of cricothyroid muscle contraction on laryngeal resistance and glottic area. Ann Otol Rhinol Laryngol. 1989;98:119.
14. Mathew OP, Abu-Osba YK, Thach BT. Influence of upper airway pressure changes in respiratory frequency. Resp Physiol. 1982;49:223.
15. Sasaki CT. Development of laryngeal function: etiologic significance in the sudden infant death syndrome. Arch Otol. 1977;103:8.
16. Korpas J, Tomori S. Cough and Other Respiratory Reflexes. Basel: Karger; 1979.
17. Garcia M. Observations of the human voice. Proc R Soc Lond. 1855;7:399.
18. Mayo H. Outlines of Human Physiology. London: Burgess & Hill; 1829.
19. Van de Graff WB. Thoracic influence on upper airway patency. J Appl Physiol. 1988;65:2124.
20. Sant’Ambrogio FB, Mathew OP, Clark WD, et al. Laryngeal influences on breathing pattern and posterior cricoarytenoid muscle activity. J Appl Physiol. 1985;58:1298.
21. Brancatisano TP, Dodd DS, Engel LA. Respiratory activity of the posterior cricoarytenoid muscle and vocal cords in humans. J Appl Physiol. 1984;57:1143.
22. Green JH, Neil E. The respiratory function of the laryngeal muscles. J Physiol Lond. 1955;129:134.
23. Mathew OP, Abu-Osba YK, Thach BT. Influence of upper airway pressure changes in genioglossus muscle respiratory activity. J Appl Physiol. 1982;52:438.
24. Bartlett D. Effects of hypercapnia and hypoxia on laryngeal resistance to airflow. Resp Physiol. 1979;37:293.
25. Murakami Y, Kirchner JA. Respiratory movements of the vocal cords: an EMG study in the cat. Laryngoscope. 1972;82:454.
26. Nakamura F, Uyeda Y, Sonoda Y. EMG study on respiratory movements of the intrinsic laryngeal muscles. Laryngoscope. 1958;68:109.
27. Sherry JH, Megirian D. Spontaneous and reflexly evoked laryngeal abductor and adductor muscle activity in cat. Exp Neurol. 1974;43:487.
28. Kuna ST, Insalaco G, Woodson GE. Thyroarytenoid muscle activity during wakefulness and sleep in normal adults. J Appl Physiol. 1988;63:1332.
29. Gautier H, Remmers JE, Bartlett DJr. Control of the duration of expiration. Resp Physiol. 1973;18:205.
30. Sant’Ambrogio G, Mathew OP, Fisher JT, et al. Laryngeal receptors responding to transmural pressure, airflow, and local muscle activity. Resp Physiol. 1983;54:317.
31. Tomori Z, Widdicombe JG. Muscular, bronchomotor and cardiovascular reflexes elicited by mechanical stimulation of the respiratory tract. J Physiol Lond. 1969;200:25.
32. Gerber U, Polosa C. Some effects of superior laryngeal nerve stimulation on sympathetic preganglionic neuron firing. Can J Physiol Pharmacol. 1979;57:1073.
33. Olson T, Woodson GE, Heldt G. Upper airway function in Ondine’s curse. Arch Otolaryngol Head Neck Surg. 1992;118:310.
34. van Lunteren E, Strohl KP. The respiratory muscles of the upper airways. Clin Chest Med. 1986;7:171-188.
35. Fritzell B. Electromyography in the study of the velopharyngeal function: a review. Folia Phoniatr (Basel). 1979;31:93.
36. Collett PW, Brancatisano AP, Engel LA. Upper airway dimensions and moments in bronchial asthma. Am Rev Respir Dis. 1986;133:1143.
37. Brouillette RT, Thach BT. A neuromuscular mechanism for maintaining extrathoracic airway patency. J Appl Physiol. 1979;46:772.
38. Remmers JE, deGroot WJ, Sauerland EK, et al. Pathogenesis of upper airway occlusion during sleep. J Appl Physiol. 1978;44:931.
39. Roberts JL, Reed WR, Thach BT. Pharyngeal airway-stabilizing function of sternohyoid and sternothyroid muscles in the rabbit. J Appl Physiol. 1984;57:1790.
40. Andrew BL. The respiratory displacement of the larynx: a study of the innervation of accessory respiratory muscles. J Physiol Lond. 1955;130:474.
41. Galen: On the Usefulness of the Parts of the Body, MT Maym trans. Ithaca. NY: Cornell University Press, 1968.
42. Husson R. Etude des phénomènes physiologiques et acoustiques fondamentaux de la voix chantée. These Fac Sc Paris. 1950.
43. Van de Berg J. Myoelastic theory of voice production. J Speech Hear Res. 1958;1:227.
44. Baer T. Investigation of the phonatory mechanism. In: Ludlow CL, editor. Proceedings of the Conference on the Assessment of Vocal Pathology, Bethesda, Maryland, April, 1979, vol 11. Rockville, Maryland: American Speech-Language-Hearing Association; 1981.
45. Hirano M, Kakita Y. Cover-body theory of vocal fold vibration. In: Daniloff RG, editor. Speech Science. San Diego, CA: College-Hill Press; 1985:1233-1268.
46. Ishizaka K, Flanagan JL. Synthesis of voiced sounds from a two-mass model of the vocal cords. Bell Syst Tech J. 1972;51:1233.
47. Hirano M. Phonosurgical anatomy of the larynx. In: Ford CN, Bless DM, editors. Phonosurgery. New York: Raven Press; 1991:26.
48. Maurer D, Hess M, Gross M. High-speed imaging of vocal fold vibrations and larynx movements within vocalizations of different vowels. Ann Otol Rhinol Laryngol. 1996;105:975.