Chapter 32 Invasive Mechanical Ventilation
Techniques
Modes of Invasive Mechanical Ventilation
Most mechanical ventilators are capable of delivering ventilation in a fully controlled manner, to assist spontaneous breathing, and to facilitate nonassisted spontaneous breathing (Figure 32-1). Normally, the means by which inhalation is terminated (cycled off) is used to classify the ventilatory modes. Common mechanisms to cycle off the inhalation include volume, pressure, airflow, and time. Initiation of an inhalation (i.e., trigger-on) can be based either on predefined time intervals or on systems in which the patient’s inspiratory effort has to exceed a specific threshold. Most frequently, pneumatic systems are used that require a predefined, adjustable change in pressure or airflow within the ventilator circuit to trigger the initiation of an inhalation by the ventilator. Alternative trigger-on and cycling-off methods, such as changes in pleural pressure (PPL) (as can be assessed by esophageal pressure [PES] measurements) or changes in the amplitude of the diaphragm electrical activity (Edi), are currently entering clinical practice.
Modes That Target Volume or Pressure
Volume-Targeted (or -Controlled) Ventilation
With time-cycled, volume-targeted (i.e., volume-controlled) ventilation (VCV), VT is preset, and the minute ventilation is guaranteed by the ventilator, depending on the ventilatory rate. Triggering delivery of additional breaths with the preset VT occurs when the patient’s breathing efforts exceed a caregiver-defined trigger-on threshold. Volume assist-control (A/C) refers to a variation of VCV in which the patient initiates delivery of a fixed VT by the ventilator, whereas a VCV backup rate ensures that the patient receives a minimum minute ventilation. Parameters that can be modified with most of the ventilators are the rate of inspiratory flow or ratio of the inspiratory to expiratory times (I/E ratio), the inspiratory rise time, the duration of the end-inspiratory pause time, and with some ventilators, the flow pattern during inhalation (Figure 32-2). The effects of changes in these parameters on the pressure and flow waveforms are demonstrated in Figure 32-2, B and C.
Pressure-Targeted (or -Controlled) Ventilation
With pressure-controlled ventilation (PCV), a maximum inspiratory pressure is targeted by the caregiver. The ventilator increases the pressure to this level during each breath. The flow pattern is decelerating; flow is high initially but decreases as the PVENT limit is approached. Inhalation is terminated when a predefined time limit or flow level is reached. The applied VT depends on the relative stiffness (elastance) of the respiratory system (e.g., VT is lower if pulmonary edema develops or if the patient contracts the abdominal or chest wall musculature) and on the resistance to airflow (e.g., VT will be lower if secretions markedly increase airway resistance). The adjustable parameters are the targeted PVENT, the inspiratory rise time, and, in some ventilators, the inspiratory time (Figure 32-3).
Modes That Deliver Assistance to Spontaneous Breathing
Patients often are ventilated with volume- or pressure-targeted modes (i.e., controlled ventilation) in the early phase of the disease process, whereas modes delivering assistance to spontaneous breathing usually are applied later. Assistance to spontaneous breathing is used to prevent respiratory muscle atrophy as well as to maintain physiologic feedback and intrinsic defense mechanisms, such as the Hering-Breuer reflex, based on the hypothesis that integration rather than abolition may help to minimize VILI. This approach should be better suited than “caregiver-controlled” mechanical ventilation to accommodate the typically rapid changes in lung mechanics and metabolic demands in critically ill patients. Ventilatory modes in which patients breathe spontaneously early in the course of the acute lung injury (ALI) process may have certain advantages, such as improved pulmonary ventilation-perfusion (V/Q) matching (Figure 32-4), increased oxygenation, preserved cardiac function, reduced need for excessive sedation, prevention of ventilation-associated respiratory muscle dysfunction, and ventilation at lower mean airway pressure, compared with controlled modes of ventilation. Induction of respiratory muscle fatigue or failure secondary to increased work of breathing is a potential disadvantage, but this frequently can be minimized with use of the appropriate level of positive end-expiratory pressure (PEEP) and adjustment of inspiratory flow rates. Of interest, recent data suggest that early neuromuscular blockade for 48 hours in patients with ALI may decrease mortality (see further on).
Pressure Support Ventilation
Pressure support ventilation is patient-triggered and, normally, flow-cycled, allowing the patient to actively control the start of each breath. Once the patient’s inspiratory effort exceeds the trigger-on threshold, a caregiver-defined level of PVENT is delivered to the airways (Figure 32-5, A). After a high initial airflow that is required to rapidly approach the targeted pressure, the airflow progressively decreases. Once a predefined percentage of the maximum inspiratory flow is reached, the ventilator terminates inhalation and opens the expiratory valve. Parameters that can be adjusted with pressure support ventilation are the trigger-on threshold, the inspiratory rise time, and the pressure level (see Figure 32-5, B). To better match termination of the inhalation with the patient’s individual demand, the cycling-off airflow threshold can be varied in some ventilators.
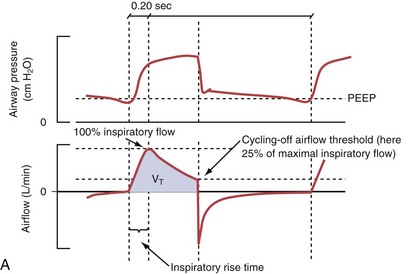
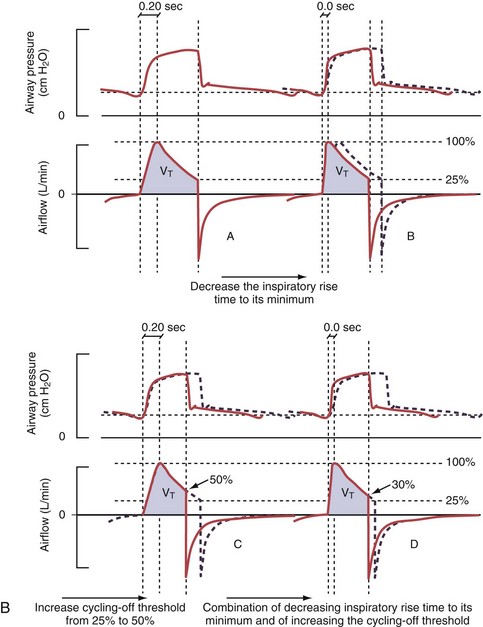
Figure 32-5 A, Pressure support ventilation (PSV). The delivered tidal volume (VT) is calculated by integrating the area under the flow curve, as indicated by the blue-shaded area. The inspiratory rise time defines how fast the maximal airflow (100%) is achieved. Thereafter, the inspiratory airflow continuously decreases because, once the pressure target is reached, maintaining this level requires progressively less air to flow into the lungs. As soon as the cycling-off airflow threshold (i.e., a preset percentage of maximal air flow) is reached, the ventilator ceases to deliver inspiratory flow, and the expiratory valve is opened to allow passive exhalation. B, Changes in the parameters of pressure support ventilation result in characteristic changes of the pressure and volume curves. Panels A to D demonstrate isolated changes of parameters assuming that all other ventilatory parameters and the mechanical characteristics of the respiratory system remain unchanged. A, PSV breath with an inspiratory rise time of 0.20 second. B, After reducing the inspiratory rise time to its minimum, the peak inspiratory flow and consequently also the cycling-off airflow threshold are both reached earlier. Decreasing the inspiratory rise time results in a shorter inspiratory time, while the VT remains unchanged. Note that although the inspiratory rise time is decreased to 0 seconds, the peak inspiratory flow is reached with a small delay. C, Increasing the cycling-off airflow threshold from 30% to 50% similarly shortens the inspiratory time; however, VT decreases in this case. D, A combination of a maximal decrease in the inspiratory time and a moderate increase in the cycling-off airflow threshold shortens the inspiratory time, whereas the loss in VT is only minimal. Such an approach can be used to achieve a prolongation of the expiratory time in patients at risk for dynamic hyperinflation because of expiratory flow limitation (e.g., patients with COPD) (see also Figure 32-19). COPD, chronic obstructive pulmonary disease.
Difficulties with Conventional Modes of Assistance to Spontaneous Breathing
Although important improvements have been made in the trigger-on characteristics and the cycling-off characteristics of ventilators, ideal synchrony between the ventilator and the patient has not been achieved with most modes of ventilation, and patient-ventilator asynchrony is common (Figure 32-6). Patient-ventilator asynchrony may result in increased inspiratory and expiratory muscle activity and may introduce an unnecessary burden, in terms of work of breathing, in patients whose respiratory muscles are already under stress. Technologies that allow delivery of assistance in proportion to the patient’s demand on a breath-by-breath basis have only recently been developed (e.g., proportional assist ventilation [PAV] and neurally adjusted ventilatory assist [NAVA], as described later on).
Variables that can be adjusted to improve synchrony between the patient and the ventilator include the trigger-on threshold, the inspiratory rise time or flow rate, and the cycling-off airflow threshold. The mechanisms used to initiate a breath (trigger-on) detect changes in airflow or pressure in the ventilatory circuit. Hence, negative deflections of short duration (pneumatic trigger mechanisms) may be detectable in the airway pressure and/or flow tracings when a breath is triggered by the patient’s effort. Delivery of the assistance is terminated either after a predefined time has elapsed (time-cycled) or after a prespecified cycling-off airflow threshold (flow-cycled) has been reached (see Figure 32-5). Rise time refers to the time required by the ventilator to increase the inspiratory airflow from zero to peak. As demonstrated in Figure 32-7, the rise time changes the slope of the increase in pressure during early inspiration. Generally, rise time (or the inspiratory flow pattern) should be set to ensure that air is delivered rapidly (fast increase in airway pressure) after initiation of a breath. By establishing an optimal inspiratory rise time, synchrony to the patient’s respiratory demand can be optimized and work of breathing can be reduced.
Proportional Assist Ventilation
PAV, the first patient-triggered mode that adapted the level of assist to the patient’s inspiratory effort, was introduced in 1987. With PAV, the ventilator delivers positive pressure throughout inspiration in proportion to the inspiratory airflow and volume generated by the patient (Figure 32-8). The magnitude of unloading is based on measuring elastance and resistance of the respiratory system. Whereas with conventional modes of ventilation the VT or the delivered PVENT is relatively constant from breath to breath, with PAV only the relationship between delivered PVENT and the inspiratory effort of the patient is constant, whereas VT and the delivered PVENT become dependent variables. Although PAV requires that the patient always assume a portion of the respiratory work, this mode has been demonstrated to effectively unload the respiratory muscles.
Neurally Adjusted Ventilatory Assist
A relatively new strategy of mechanical ventilation, NAVA, uses the Edi to control the ventilator (Figure 32-9). Because breathing signals originate from the brain and reach the diaphragm by way of the phrenic nerves, Edi represents the neural respiratory effort with respect to both timing and amplitude. During NAVA, positive pressure is applied to the airway opening in direct proportion to the Edi amplitude, so defining a target pressure or volume is not required. The patient’s respiratory control mechanisms, including feedback from mechanoreceptors and chemoreceptors, adjust the Edi and thereby regulate the pressure and delivered volume. Animal data and a number of clinical studies suggest that NAVA is applicable in the ICU environment, efficiently delivers assistance synchronous to the subject’s demand, unloads the respiratory muscles, maintains gas exchange, and preserves cardiac performance during invasive ventilation and also during noninvasive ventilation even with use of an excessively leaky interface. Simultaneous measurement of the Edi (which reflects the patient’s neurally generated effort) and the delivered assist allows monitoring the patient’s ability to translate a neural effort into ventilation, referred to as neuroventilatory efficiency. NAVA does not require measurement of respiratory system mechanics, and runaway phenomena are unlikely to occur.
Combined Modes
Airway Pressure Release Ventilation
With airway pressure release ventilation (APRV) (Figure 32-10), the pressure in the ventilator circuit alternates between a high and a lower level (normally the higher pressure level is of longer duration than the lower pressure level) and spontaneous breathing is allowed in any phase of the cycle. The high- and low-pressure levels, the rate of change between the two levels, the respiratory system compliance, and the airway resistance to flow are the main determinants of the “mechanical ventilation” portion with APRV, whereas the complementary “spontaneous breathing” portion mainly depends on the patient’s respiratory drive. In contrast with continuous positive airway pressure (CPAP), APRV interrupts PVENT briefly to augment spontaneous minute ventilation and thereby increases alveolar ventilation and CO2 removal without increasing the work of breathing. Spontaneous efforts during APRV are not actively assisted except for those breaths that happen to occur during the change from the lower to the upper pressure level. Total minute ventilation with APRV is the sum of the mechanical, pressure-controlled ventilation and the complementary spontaneous breathing. APRV without spontaneous breathing is essentially the same as PCV.
Bilevel Positive Airway Pressure Ventilation
With BiPAP ventilation, two levels of continuous positive pressure are used, as with APRV, and unrestricted spontaneous breathing is allowed on both pressure levels (Figure 32-11, A). As an additional option, assistance to spontaneous breathing efforts can be provided at the lower pressure level (see Figure 32-11, B), at the higher pressure level, or at both levels. The transition from the low-pressure to the high-pressure level is coordinated with the patient’s breathing effort. The designations Bi-Vent, DuoPAP, and Bi-level used by ventilator manufacturers are synonymous with BiPAP.
Synchronized Intermittent Mandatory Ventilation
Synchronized intermittent mandatory ventilation (SIMV) combines volume- or pressure-targeted breaths at a caregiver-defined rate (mandatory breaths) with unassisted spontaneous breathing (Figure 32-12). Because ideally the mandatory breaths should be synchronized to the patient’s own breathing effort, SIMV requires that the ventilator settings for the trigger-on threshold be adequate for this purpose.
Modes That Facilitate Spontaneous Breathing
Continuous Positive Airway Pressure
With CPAP, a caregiver-defined level of positive pressure is maintained by the ventilator while the patient is breathing spontaneously (Figure 32-13). Of note, because the patient’s breathing efforts are not assisted with CPAP, the patient must have adequate respiratory drive and adequate respiratory muscle function. CPAP helps to increase and maintain the functional residual capacity (FRC) and thereby to increase the lung units available for gas exchange (reduction of intrapulmonary right-to-left shunt), prevent end-expiratory airway collapse, and counter the effects of auto-PEEP or intrinsic PEEP.
Positive End-Expiratory Pressure
Given the current lack of tools to monitor both alveolar overdistention and collapse, and given the heterogeneity of the distribution of most disease processes within the lung, identification of the “best PEEP?” level is not straightforward in clinical practice. In fact, the gravitational gradient in pleural pressure and end-expiratory lung volume that exists in both normal subjects and patients requiring mechanical ventilation implies that there cannot be a single level of PEEP that is “best” for all regions of the lung. Pending further clarification on how to set PEEP, a pragmatic approach for daily clinical practice in patients with ALI is to adhere to the algorithm used in the large Acute Respiratory Distress Syndrome Network (ARDSNet) trial (Table 32-1) and to carefully observe the effect of changes in PEEP on parameters such as blood oxygenation, cardiac performance, and expiratory flow limitation. Of note, in patients with stiff chest walls (e.g., massive ascites), higher levels of PEEP are warranted.
Table 32-1 Combinations of Inspiratory Oxygen Fraction (FIO2) and Positive End-Expiratory Pressure (PEEP) in Patients with Acute Lung Injury or Acute Respiratory Distress Syndrome to Achieve Oxygenation Goals*
FIO2 | PEEP (cm H2O) |
---|---|
0.3 | 5 |
0.4 | 5 |
0.4 | 8 |
0.5 | 8 |
0.5 | 10 |
0.6 | 10 |
0.7 | 10 |
0.7 | 12 |
0.7 | 14 |
0.8 | 14 |
0.9 | 14 |
0.9 | 16 |
0.9 | 18 |
1.0 | 18 |
1.0 | 20 |
1.0 | 22 |
1.0 | 24 |
* Partial pressure of arterial oxygen (PaO2) of 55 to 80 mm Hg or oxyhemoglobin saturation measured by pulse oximetry (SpO2) of 88% to 95%.
Modified from Ventilation with lower tidal volumes as compared with traditional tidal volumes for acute lung injury and the acute respiratory distress syndrome. The Acute Respiratory Distress Syndrome Network, N Engl J Med 342:1301–1308, 2000.
Principles of Respiratory System Mechanics Relevant to Mechanical Ventilation
Airway Resistance and Lung Elastance
To a simplified approximation, the patient-ventilator unit can be considered as an in-series mechanical system that consists of a resistive element (ventilator and endotracheal tubing + central airways) and an elastic element (lung-thorax compartment). During inflation, the pressure applied to the tube inlet (PVENT) is equal to the sum of the pressure required to overcome the resistive elements (PRESIST) and the pressure required to distend the lung and chest wall (PELAST). The flow through the resistive element is a function of the difference in pressure between the tube inlet and the tube outlet (PRESIST) and the resistance of the tubing system (i.e., flow [L/min] = PRESIST [cm H2O]/resistance [cm H2O × min/L]). For example, forcing air at a low flow rate through a large-bore tube requires less pressure than if a high flow is applied to a small bore tube. Because PRESIST is used to overcome the resistive element, only PELAST is applied across the respiratory system (i.e., the lungs and the chest wall). Figure 32-14 demonstrates the relationship between PVENT and the pressure within the central airways (PAW) throughout a respiratory cycle.
The relative stiffness (or elastance) of the lung and the chest wall define what proportion of PAW is used to distend the chest wall and what proportion is used to distend the lung (Figure 32-15). For example, if the elastance of the chest wall is twice that of the lung, then two thirds of PAW is used to distend the chest wall and only one third is used to distend the lung. The fractions EL/ERS and ECW/ERS determine how PAW is apportioned between the lung (PL = PAW × EL/ERS) and the chest wall (PCW = PAW × ECW/ERS). Predicting PL on the basis of PAW without information on the elastance of the lung and the chest wall is not possible. Furthermore, lung and chest wall elastance vary among individuals and may also change over time during critical illness (e.g., with accumulation of edema or ascites). Although calculating the elastance of the entire respiratory system (ERS) is relatively easy (ERS = PVENT/VT), calculating the elastance of the lung and the chest wall separately is unfortunately not straightforward and requires knowledge of PL. Figure 32-16 illustrates the intrabreath changes in PL during unassisted spontaneous breathing and during volume-targeted ventilation.
Dynamic Hyperinflation and Auto-Peep
Classically, dynamic hyperinflation is present in patients with COPD, in whom the unstable airways collapse during exhalation (Figure 32-17), and in patients with asthma, in whom increased bronchomotor tone impedes exhalation. Exacerbation of the disease process such as bronchospasm in asthma or bronchitis with thickening of the mucosa in COPD worsens the condition. Of note, auto-PEEP also may develop in patients with more restrictive disease processes such as ARDS, in which intrapulmonary time constants are widely inhomogeneous, or when low VT settings at high ventilatory rates are used. A narrow-diameter or kinked endotracheal tube, inspissated secretions, an obstructed filter in the expiratory limb of the ventilatory circuit, a highly variable respiratory rate, or tachypnea will further predispose the respiratory system to development of auto-PEEP.
Persistent airflow at the end of exhalation, especially in combination with consistent failure to trigger the ventilator with inspiratory efforts, should heighten clinical suspicion for the presence of dynamic hyperinflation. Measurement of auto-PEEP requires equilibration of the pressure across the entire lung during occlusion of the expiratory valve at end-expiration (Figure 32-18), ideally performed during muscle paralysis (but paralysis usually should not be undertaken solely to make this measurement). Measurement of auto-PEEP during spontaneous breathing is difficult and often unreliable, because the inspiratory and expiratory efforts interfere with the procedure, and studies have shown that expiratory muscle contraction can occur. These contractions may be difficult to detect clinically.
Dynamic hyperinflation can markedly increase the oxygen cost of breathing in a spontaneously breathing patient (Figure 32-19). Because the compliance of the respiratory system is lower at high lung volumes, more energy is required to expand the lungs. Furthermore, with dynamic hyperinflation the patient needs to produce large pleural pressure swings to overcome the auto-PEEP before pressure in the ventilator circuit decreases below the applied PEEP level and before pneumatic trigger systems located in the ventilator can be excited. Because generation of force by the inspiratory muscles is impaired during hyperinflation (decreased resting length of the diaphragm requires a higher-than-normal respiratory drive to lower pleural pressure), triggering the ventilator becomes challenging for patients with COPD and especially for those who have weakness or fatigue of the respiratory muscles—both of these conditions are difficult to distinguish from the effects of trying to inhale while breathing at a lung volume near total lung capacity (TLC).
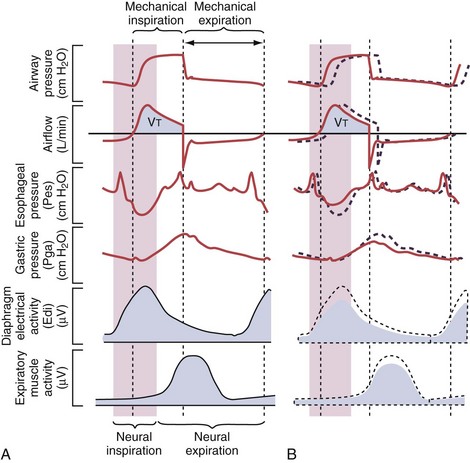
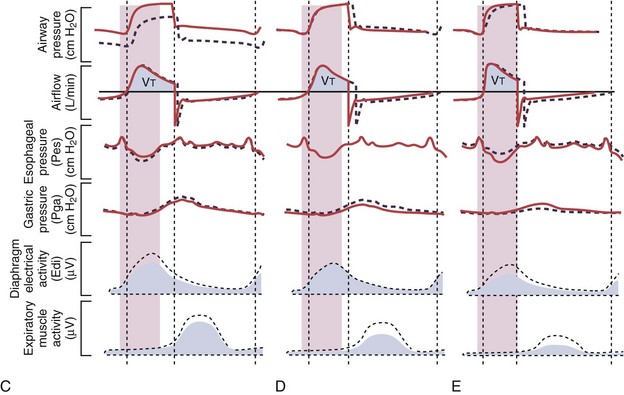
Figure 32-19 A, Demonstration of delivery of assist with pressure support ventilation (PSV) in a patient with expiratory flow limitation resulting in dynamic hyperinflation. There is substantial asynchrony (delayed triggering-on and cycling-off) between the assist delivered by the ventilator and the patient’s (neural) respiratory demand, as reflected by the electrical activity of the diaphragm (Edi). The high amplitudes for the Edi and for esophageal pressure (Pes) deflections indicate that the inspiratory muscles are highly active during delivery of pressure by the ventilator, whereas the high amplitudes for the expiratory muscle activity and for the gastric pressure (Pga) deflections indicate that the patient uses his expiratory muscles to counter delivery of pressure by the ventilator during neural expiration. B, After optimizing the trigger-on threshold, delivery of assist starts earlier (requiring less inspiratory effort) and also ceases earlier (requiring less activation of the expiratory muscles). C, Adjusting the level of extrinsic PEEP to compensate for auto-PEEP allows earlier detection of the inspiratory effort by the ventilator (see Figure 32-21) and helps to further reduce the inspiratory workload. D, Increasing the cycling-off airflow threshold results in earlier termination of the assist. Hence, expiratory muscle activity can be reduced. Note that the ventilator inspiratory time, as well as the delivered tidal volume (VT), decreases when the cycling-off airflow threshold is increased, whereas the expiratory time increases (provided the respiratory rate remains unchanged). E, When the inspiratory rise time is reduced, the peak inspiratory flow and thus the cycling-off airflow threshold both are reached earlier, and the inspiratory time is further shortened. After completion of all steps as demonstrated here (B to E), ventilator assist is delivered in synchrony with the patient’s neural respiratory demand, and unloading of the inspiratory muscles is achieved as reflected by minimization of the amplitudes for Edi and for Pes deflections. Expiration is driven only by the elastic recoil of the lung and the chest wall, as reflected by minimization of the amplitudes for expiratory muscle activity and for Pga deflections. PEEP, positive end-expiratory pressure.
Inappropriate settings during mechanical ventilation can worsen dynamic hyperinflation, especially when high ventilatory rates and/or high VT settings resulting in excessive minute ventilation are used, when the assist is delivered asynchronous to the patient’s demand (Figure 32-20), or when PEEP levels higher than those needed to counterbalance auto-PEEP are used.
The first approach to minimizing dynamic hyperinflation in a patient with obstructive airway disease is to decrease the resistance in the expiratory airways by removing any mechanical obstruction and by treating bronchospasm and airway inflammation. The most effective and abrupt way to decrease auto-PEEP is to reduce minute ventilation, although this may lead to an increase in an already elevated arterial PCO2 (PaCO2). Alternatively, adding extrinsic PEEP (Figure 32-21) will decrease the work of breathing, thereby reducing CO2 production and lowering the PaCO2 even if alveolar ventilation is unchanged. Because patients with COPD with chronically elevated PaCO2 levels retain sufficient bicarbonate to normalize arterial pH, minute ventilation should not be adjusted to maintain a normal PaCO2. In addition, the inspiratory phase should be shortened (thereby allowing maximum time for exhalation), as demonstrated in Figures 32-19 and 32-20. Of note, the variability in the duration of the expiratory phase and, hence, in the expired volume per breath increases when switching from a controlled mode of ventilation to a mode that delivers assistance to spontaneous breathing. This may result in modification of the degree of dynamic hyperinflation on a breath-by-breath basis and can induce patient-ventilator asynchrony because of wasted inspiratory efforts, especially when high levels of assist are used (see Figure 32-6).
Ventilator-Induced Lung Injury
Diseased lungs are more susceptible than healthy lungs to the development of VILI. VILI also can initiate and propagate cascades (e.g., upregulation of a systemic inflammatory response) that ultimately culminate in multiple system organ failure (MSOF) (Figure 32-22). A ventilatory strategy that uses low VT and limited PVENT is not only protective to the lung but also has the potential to reduce the incidence of MSOF. Exposure to excessive mechanical stresses can result in damage to lung tissue and cell integrity from either of two primary factors: (1) overdistention of the lung (i.e., volutrauma) and (2) repetitive air space recruitment and derecruitment (atelectrauma). The critical feature defining induction of VILI secondary to volutrauma seems to be the degree of regional lung distention, rather than the absolute PVENT reached. High pressures per se in the respiratory system do not necessarily result in VILI. For example, trumpet players repeatedly generate very high airway pressures (more than 150 cm H2O) without incurring lung damage, because no excessive lung distention occurs.
Strategies to Prevent Ventilator-Induced Lung Injury in Clinical Practice
The beneficial effects of using relatively low VT, and possibly also higher levels of PEEP, probably are related to the two main components—reduction of VILI and prevention of nonpulmonary organ dysfunction—as suggested by many preclinical and smaller clinical studies. In support of this mechanism, a recent large clinical study by Mascia and colleagues demonstrated that a lung-protective ventilatory strategy using VT of 6 to 8 mL/kg PBW combined with higher levels of PEEP prevented the decline of pulmonary function in brain-dead organ donors and roughly doubled the number of lungs available for transplantation. Another recent large clinical study demonstrated that pharmacologic neuromuscular blockade for 48 hours early in the course of ARDS resulted in both better survival and less time spent on the ventilator, compared with the use of placebo. Possible mechanisms by which neuromuscular blockade might lead to improved outcome are summarized in Figure 32-23.
Pending further clarification of how to define optimal PEEP levels in individual patients, a pragmatic approach for daily clinical practice is to adhere to the algorithm used in the large ARDSNet trial (see Table 32-1). Determining optimal ventilator setting in an individual patient with ALI or ARDS is always a continuous, iterative process of evaluation, intervention, and reevaluation that must take into account changes in the disease process over time.
Alternative Approaches to Lung Recruitment
HFV encompasses a number of ventilatory modes, including high-frequency positive-pressure ventilation (HFPPV), high-frequency jet ventilation (HFJV), high-frequency flow interruption (HFFI), and high-frequency oscillatory ventilation (HFOV), all of which use substantially higher ventilatory frequencies (i.e., in the range of 1 to 25 Hz) and much lower VT than with conventional modes (Figure 32-24). During HFV, the VT typically is less than the dead space. Gas transport is accomplished by various aspects of convection and diffusion. With HFV, a high mean PVENT is used to recruit alveoli and maintain lung volume above FRC. Thus, in contrast with controlled modes of ventilation, HFV maintains lung volume at a relatively constant level and uses very small VT to accomplish ventilation. Intermittent sighs or sustained inflations are optionally used to recruit collapsed lung regions and to avoid atelectasis.
Weaning from Mechanical Ventilation
Good clinical judgment in this regard is essential: Only patients with a reasonable likelihood of being able to breathe on their own are suitable candidates for attempts at weaning. Although measuring a variety of physiologic variables may help guide this decision, the process often entails a “trial and error” component. Careful monitoring of the patient’s comfort, gas exchange, respiratory mechanics, and hemodynamics during a trial of spontaneous breathing is mandatory. The protocol-based use of spontaneous breathing trials (SBTs) is recommended to identify patients who are likely to be able to breathe spontaneously without assistance (Figure 32-25). Criteria to initiate an SBT as recently recommended by a consensus conference are summarized in Box 32-1 (for details, see the cited source paper authored by MacIntyre and co-workers), but each patient must be evaluated for specific factors that might modify the recommendation or mandate an alternate approach.
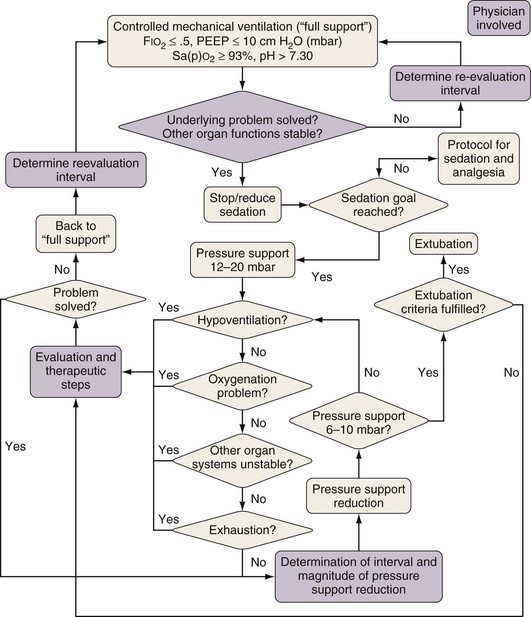
Figure 32-25 Example of a protocol for gradual reduction in the assist level during weaning.
(Modified from Jakob SM, Lubszky S, Friolet R, et al: Sedation and weaning from mechanical ventilation: effects of process optimization outside a clinical trial, J Crit Care 22:219–228, 2007.)
Box 32-1
Criteria for Initiation of a Spontaneous Breathing Trial*
Evidence of some reversal of the underlying cause of respiratory failure
Adequate oxygenation: PaO2/FIO2 ratio ≥150-200, required PEEP ≤5-8 cm H2O, FIO2 ≤0.4-0.5, and pH ≥7.25
Hemodynamic stability as defined by the absence of active myocardial ischemia and the absence of clinically significant hypotension—that is, a condition requiring no vasopressor therapy or therapy with only low-dose vasopressors (e.g., dopamine or dobutamine given at a dose of less than 5 µg/kg/minute).
Modified from MacIntyre NR, Cook DJ, Ely EW Jr, et al: Evidence-based guidelines for weaning and discontinuing ventilatory support: a collective task force facilitated by the American College of Chest Physicians; the American Association for Respiratory Care; and the American College of Critical Care Medicine, Chest 120(suppl):375–395, 2001.
A formal SBT often is not required after short-term ventilation (e.g., in patients ventilated for less than 24 hours as, for example, in the postoperative period), whereas an SBT should be performed on a daily basis during a daily interruption of sedation in those patients who meet certain criteria (see Box 32-1). Conventionally, an SBT is performed with use of a minimal level of assist (i.e., 0 to 7 cm H2O, preferably 0 cm H2O), an FIO2 of 0.5, and a PEEP level of 5 to 7.5 cm H2O. An initial brief period of spontaneous breathing can be used to assess the advisability of continuing on to a formal SBT. The criteria used to assess the patient’s readiness to continue tolerance during SBTs are the respiratory pattern, the adequacy of gas exchange, hemodynamic stability, and subjective comfort. Tolerating an SBT for 30 to 120 minutes warrants discontinuation of the ventilator. Removal of the artificial airway is a separate consideration and is based on assessing airway patency and the ability of the patient to protect the airway.
When an SBT fails, the cause should be determined. Once reversible causes for failure are corrected, and if the patient still meets the criteria listed in Box 32-1, subsequent SBTs should be performed at least every 24 hours. Patients who fail an SBT should receive a stable, nonfatiguing, comfortable form of ventilatory support. Anesthesia and sedation strategies and ventilator management aimed at early extubation should be used in postsurgical patients. Weaning protocols designed for nonphysician health care professionals should be developed and implemented by intensive care units (ICUs). Protocols aimed at optimizing sedation also should be developed and implemented.
Briel M, Meade M, Mercat A, et al. Higher vs lower positive end-expiratory pressure in patients with acute lung injury and acute respiratory distress syndrome: systematic review and meta-analysis. JAMA. 2010;303:865–873.
dos Santos CC, Slutsky AS. The contribution of biophysical lung injury to the development of biotrauma. Annu Rev Physiol. 2006;68:585–618.
Fan E, Needham DM, Stewart TE. Ventilatory management of acute lung injury and acute respiratory distress syndrome. JAMA. 2005;294:2889–2896.
Gattinoni L, Caironi P. Refining ventilatory treatment for acute lung injury and acute respiratory distress syndrome. JAMA. 2008;299:691–693.
Gattinoni L, Caironi P, Cressoni M, et al. Lung recruitment in patients with the acute respiratory distress syndrome. N Engl J Med. 2006;354:1775–1786.
Jaber S, Petrof BJ, Jung B, et al. Rapidly progressive diaphragmatic weakness and injury during mechanical ventilation in humans. Am J Respir Crit Care Med. 2011;183:364–371.
Levine S, Nguyen T, Taylor N, et al. Rapid disuse atrophy of diaphragm fibers in mechanically ventilated humans. N Engl J Med. 2008;358:1327–1335.
MacIntyre NR, Cook DJ, Ely EWJr, et al. Evidence-based guidelines for weaning and discontinuing ventilatory support: a collective task force facilitated by the American College of Chest Physicians; the American Association for Respiratory Care; and the American College of Critical Care Medicine. Chest. 2001;120(suppl):375–395.
Mascia L, Pasero D, Slutsky AS, et al. Effect of a lung protective strategy for organ donors on eligibility and availability of lungs for transplantation: a randomized controlled trial. JAMA. 2010;304:2620–2627.
Papazian L, Forel JM, Gacouin A, et al. Neuromuscular blockers in early acute respiratory distress syndrome. N Engl J Med. 2010;363:1107–1116.
Sud S, Friedrich JO, Taccone P, et al. Prone ventilation reduces mortality in patients with acute respiratory failure and severe hypoxemia: systematic review and meta-analysis. Intensive Care Med. 2010;36:585–599.
Tobin MJ, Laghi F, Jubran A. Ventilator-induced respiratory muscle weakness. Ann Intern Med. 2010;153:240–245.
Tremblay LN, Slutsky AS. Ventilator induced lung injury: from the bench to the bedside. Intensive Care Med. 2006;32:24–33.
2000 Ventilation with lower tidal volumes as compared with traditional tidal volumes for acute lung injury and the acute respiratory distress syndrome. Acute Respiratory Distress Syndrome Network. N Engl J Med. 2000;342:1301–1308.