CHAPTER 37 Inner ear
The inner ear contains the organ of hearing, the cochlea, and the organs of balance, the utricle, saccule and semicircular canals. It consists of the bony (osseous) labyrinth, a series of interlinked cavities in the petrous temporal bone, and the membranous labyrinth of interconnected membranous sacs and ducts that lie within the bony labyrinth. The gap between the internal wall of the bony labyrinth and the external surface of the membranous labyrinth is filled with perilymph, a clear fluid with an ionic composition similar to that of other extracellular fluids, i.e. low in potassium ions and high in sodium and calcium. The membranous labyrinth contains endolymph, a fluid with an ionic composition more like that of cytosol, i.e. high in potassium ions and low in sodium and calcium. Moreover, the endolymphatic compartment is approximately 80 mV more positive than the perilymphatic compartment. These differences in ionic composition and potential are essential to the primary function of the inner ear because they provide the driving force for mechanotransduction, the process by which sensory hair cells convert the vibrations set up in the inner ear fluids by head or sound movements into electrical signals that are transmitted via the vestibulocochlear nerve to the vestibular and cochlear nuclei respectively in the brain stem.
The disarticulated temporal bone is described in detail in Chapter 36. The internal acoustic meatus and bony labyrinth are described here.
OSSEOUS (BONY) LABYRINTH
The bony labyrinth lies within the petrous part of the temporal bone. It consists of the vestibule, semicircular canals and cochlea, which are all cavities lined by periosteum and which contain the membranous labyrinth (Fig. 37.1; see Fig. 37.3A). The bone is denser and harder than that of the other parts of the petrous bone, and it is therefore possible, particularly in young skulls, to dissect the bony labyrinth out from the petrous temporal bone.
VESTIBULE
The vestibule is the central part of the bony labyrinth and lies medial to the tympanic cavity, posterior to the cochlea and anterior to the semicircular canals (Figs 37.1 and 37.2). It is somewhat ovoid in shape but flattened transversely, and (on average) measures 5 mm from front to back and vertically, and 3 mm across. In its lateral wall is the opening of the oval window (fenestra vestibuli) into which the base of the stapes inserts, and to which the base of the stapes is attached by an anular ligament. Anteriorly, on the medial wall, is a small spherical recess that contains the saccule; it is perforated by several minute holes, the macula cribrosa media, which transmit fine branches of the vestibular nerve to the saccule. Behind the recess is an oblique vestibular crest, the anterior end of which forms the vestibular pyramid. This crest divides below to enclose a small depression, the cochlear recess, which is perforated by vestibulocochlear fascicles as they pass to the vestibular end of the cochlear duct. Posterosuperior to the vestibular crest, in the roof and medial wall of the vestibule, is the elliptical recess (Fig. 37.1B), which contains the utricle. The pyramid and adjoining part of the elliptical recess are perforated by a number of holes, the macula cribrosa superior. The holes in the pyramid transmit the nerves to the utricle and those in the recess transmit the nerves to the ampullae of the superior and lateral semicircular canals (Fig. 37.1B). The region of the pyramid and elliptical recess corresponds to the superior vestibular area in the internal acoustic meatus (see Fig. 37.4). The vestibular aqueduct opens below the elliptical recess. It reaches the posterior surface of the petrous bone and contains one or more small veins and part of the membranous labyrinth, the endolymphatic duct (see Fig. 37.3A). In the posterior part of the vestibule are the five openings of the semicircular canals; in its anterior wall is an elliptical opening that leads into the scala vestibuli of the cochlea.
SEMICIRCULAR CANALS
The three semicircular canals, anterior (superior), posterior and lateral (horizontal), are located posterosuperior to the vestibule (Fig. 37.1; see Fig. 37.3). They are compressed from side to side and each forms approximately two-thirds of a circle. They are unequal in length, but similar in diameter along their lengths, except where they bear a terminal swelling, an ampulla, which is almost twice the diameter of the canal.
The anterior semicircular canal is 15–20 mm long. It is vertical in orientation and lies transverse to the long axis of the petrous temporal bone under the anterior surface of its arcuate eminence. The eminence may not accurately coincide with this semicircular canal, but may instead be adapted to the occipitotemporal sulcus on the inferior surface of the temporal lobe of the brain. The ampulla at the anterior end of the canal opens into the upper and lateral part of the vestibule. Its other end unites with the upper end of the posterior canal to form the crus commune (common limb), which is 4 mm long, and opens into the medial part of the vestibule.
The two lateral semicircular canals of the two ears are often described as being in the same plane and the anterior canal of one side as being almost parallel with the opposite posterior canal. However, measurements of the angular relations of the planes of the semicircular osseous canals in 10 human skulls led Blanks et al (1975) to suggest that the planes of the three ipsilateral canals are not completely perpendicular to each other. The angles were measured as: horizontal/anterior 111.76 ± 7.55°, anterior/posterior 86.16 ± 4.72°, posterior/horizontal 95.75 ± 4.66°. The planes of similarly orientated canals of the two sides also showed some departure from being parallel: left anterior/right posterior 24.50 ± 7.19°, left posterior/right anterior 23.73 ± 6.71°, left horizontal/right horizontal 19.82 ± 14.93°. The same observers (Curthoys et al 1977) also measured the dimensions and radii of the canals. The means for the radii of the osseous canals were found to be as follows: horizontal 3.25 mm, anterior 3.74 mm, posterior 3.79 mm. The diameters of the osseous canals are 1 mm (minor axis) and 1.4 mm (major axis). The membranous ducts within them are much smaller, but are also elliptical in transverse section, and have major and minor axes of 0.23 and 0.46 mm (see Fig. 37.3B). Representative means for ampullary dimensions are as follows: length 1.94 mm, height 1.55 mm. Phylogenetic studies suggest that the arc sizes of the semicircular canals in humans and other primates may be functionally linked to sensory control of body movements. The angulation and dimensions of the canals may be related to locomotor behaviour and possibly to agility, or more specifically to the frequency spectra of natural head movements (see review by Spoor & Zonneveld 1998).
COCHLEA
The cochlea (from the Greek cochlos for snail) is the most anterior part of the labyrinth, lying in front of the vestibule (Figs 37.1 and 37.2A; see Fig. 37.3A). It is 5 mm from base to apex, and 9 mm across its base. Its apex, or cupula, points towards the anterosuperior area of the medial wall of the tympanic cavity (Fig. 37.2A). Its base faces the bottom of the internal acoustic meatus and is perforated by numerous apertures for the cochlear nerve. The cochlea has a conical central bony core, the modiolus, and a spiral canal runs around it. A delicate osseous spiral lamina (or ledge) projects from the modiolus, partially dividing the canal (Fig. 37.2B). Within this bony spiral lies the membranous cochlear duct, attached to the modiolus at one edge and to the outer cochlear wall by its other edge. There are therefore three longitudinal channels within the cochlea. The middle canal (the cochlear duct or scala media) is blind, and ends at the apex of the cochlea; its flanking channels communicate with each other at the modiolar apex at a narrow slit, the helicotrema (Fig. 37.3A). Two elastic membranes form the upper and lower bounds of the scala media. One is Reissner’s membrane, the thin vestibular membrane that separates the scala media from the scala vestibuli. The other is the basilar membrane, which forms the partition between the scala media and the scala tympani. The organ of Corti, the sensory epithelium underlying hearing, sits on the inner surface of the basilar membrane. At the base of the scala vestibuli is the oval window (fenestra vestibuli), which leads onto the vestibular cavity but is sealed by the footplate of the stapes. The scala tympani is separated from the tympanic cavity by the secondary tympanic membrane at the round window (fenestra cochleae). The central cochlear core, the modiolus, has a broad base near the lateral end of the internal acoustic meatus, where it corresponds to the spiral tract (tractus spiralis foraminosus). There are several openings in this area for the fascicles of the cochlear nerve: those for the first 1½ turns run through the small holes of the spiral tract, and those for the apical turn run through the hole that forms the centre of the tract. Canals from the spiral tract go through the modiolus and open in a spiral sequence into the base of the osseous spiral lamina. Here the small canals enlarge and fuse to form Rosenthal’s canal, a spiral canal in the modiolus which follows the course of the osseous spiral lamina and contains the spiral ganglion (Fig. 37.2B). The main tract continues through the centre of the modiolus to the cochlear apex.
The osseous or primary spiral lamina is a ledge that projects from the modiolus into the osseous canal like the thread of a screw (Fig. 37.2B). It is attached to the inner edge of the basilar membrane and ends in a hook-shaped hamulus at the cochlear apex, partly bounding the helicotrema (Fig. 37.3A), which is an opening connecting the scala tympani and scala vestibuli. From Rosenthal’s canal, many tiny canals, the habenula perforata, radiate through the osseous lamina to its rim; they carry fascicles of the cochlear nerve to the organ of Corti. A secondary spiral lamina projects inwards from the outer cochlear wall towards the osseous spiral lamina and is attached to the outer edge of the basilar membrane. It is most prominent in the lower part of the first turn: the gap between the two laminae increases progressively towards the cochlear apex, which means that the basilar membrane is wider at the apex of the cochlea than at the base.
MICROSTRUCTURE OF THE BONY LABYRINTH
The wall of the bony labyrinth is lined by fibroblast-like perilymphatic cells and extracellular fibres (Fig. 37.3B). The morphology of the cells varies in different parts of the labyrinth. Where the perilymphatic space is narrow, as in the cochlear aqueduct, the cells are reticular or stellate in form, and give off sheet-like cytoplasmic extensions that cross the space. Where the space is wider, as in the scalae vestibuli and tympani of the cochlea and much of the vestibule, the perilymphatic cells on the periosteum and the external surface of the membranous labyrinth are extremely flat, and resemble a squamous epithelium. Elsewhere, on parts of the perilymphatic surface of the basilar membrane, the cells are cuboidal. Bundles of collagen fibres are closely related to the periosteal and labyrinthine aspects of these cells.
Recent evidence suggests that micropores or canaliculi (canaliculi perforantes) (0.2–23.0 μm diameter) are more widely distributed within the bony surfaces lining the perilymphatic space than was previously suspected: they are numerous in the peripheral and modiolar portions of the osseous spiral lamina and the floor of the scala tympani, but sparse in the osseous wall of the scala vestibuli. The proposal that these canaliculi normally provide an extensive fluid communication channel between the scala tympani and the spiral canal of the cochlea could have implications not only for novel drug-based cochlear therapies delivered via the scala tympani and the delivery of stem cells or appropriate cell lines into the deafened cochlea, but also for the design of implanted perimodiolar electrode arrays (Shepherd & Colreavy 2004). (For further reading about the changes in the inner ear that are induced by implanted cochlear electrodes, both acute and long term, see Kiefer et al 2006.)
Composition of inner ear fluids
The space between the bony and membranous labyrinths is filled with perilymph (Fig. 37.3B). The membranous labyrinth is filled with endolymph, a fluid produced by the marginal cells of the stria vascularis and the dark cells of the vestibule (see review by Wangemann & Schacht 1996) (Fig. 37.2B). Whatever their relative contributions, endolymph probably circulates in the labyrinth; it enters the endolymphatic sac, where it is transferred into the adjacent vascular plexus via the specialized epithelium of the sac. Pinocytotic removal of fluid may also occur in other labyrinthine regions.
Perilymph was initially considered to be an ultrafiltrate of plasma because of its low protein content. However, it more closely resembles cerebrospinal fluid in ionic composition, particularly in the scala tympani. Its composition is not precisely the same in both cochlear scalae: concentrations of potassium, glucose, amino acids and proteins are greater in the scala vestibuli. This has led to the suggestion that perilymph in the scala vestibuli is derived from plasma via the endothelial boundary of the cochlear blood vessels, whereas the perilymph in the scala tympani contains some cerebrospinal fluid derived from the subarachnoid spaces via the cochlear canaliculus. However, the lack of significant bulk flow suggests that perilymph homeostasis is predominantly locally regulated. Perilymph contains approximately 5 mM K+, 150 mM Na+, 120 mM Cl− and 1.5 mM Ca2+. Endolymph contains greater K+ (150 mM) and Cl− (130 mM) concentrations and lower Na+ (2 mM) and Ca2+ (20 μM) concentrations than perilymph. The major differences in ionic composition between the two fluids are important for the function of the inner ear. Displacements of the stereociliary bundles of the sensory cells activate relatively non-specific cationic channels in the stereociliary tips which allow an influx of cations, particularly K+ and Ca2+, from the endolymph. Hair cells also possess K+ channels activated by membrane voltage or intracellular Ca2+ concentrations, and these allow efflux of K+ into the perilymph which bathes their basal and lateral membranes. In addition, synaptic transmission at the base and sides of hair cells depends on the influx of Ca2+ from the perilymph through voltage-dependent calcium channels in order to release neurotransmitter.
INTERNAL ACOUSTIC MEATUS
The internal acoustic meatus is separated from the internal ear at its lateral fundus by a vertical plate divided unequally by a transverse crest (Fig. 37.4). The canal for the facial nerve passes above and anterior to the crest. Posterior to the crest, the superior vestibular area contains openings for nerves to the utricle and anterior and lateral semicircular ducts. Below the crest, an anterior cochlear area contains a spiral of small holes, the tractus spiralis foraminosus, which encircles the central cochlear canal. Behind this, the inferior vestibular area contains openings for saccular nerves, and most posteroinferior, a single hole (foramen singulare) admits the nerve to the posterior semicircular duct.
MEMBRANOUS LABYRINTH
The membranous labyrinth is separated from the periosteum by a space that contains perilymph and a web-like network of fine blood vessels (Fig. 37.3). It can be divided into two major regions, the vestibular apparatus and the cochlear duct.
VESTIBULAR APPARATUS
Utricle
The utricle is the larger of the two major vestibular sacs. It is an irregular, oblong, dilated sac that occupies the posterosuperior region of the vestibule (Fig. 37.3A), and contacts the elliptical recess (where it is a blind-ended pouch) and the area inferior to it.
The macula of the utricle (or utriculus) is a specialized area of neurosensory epithelium lining the membranous wall, and is the largest of the vestibular sensory areas (Fig. 37.5). It is triangular or heart-shaped in surface view and lies horizontally with its long axis orientated anteroposteriorly and its sharp angle pointing posteriorly (Fig. 37.6). It is flat except at the anterior edge, where it is gently folded in on itself, and it measures 2.8 mm long by 2.2 mm wide. The mature form of the macula is reached early in development, but in the adult a bulge is often present on the anterolateral border; there is sometimes an indentation at the anteromedial border. The epithelial surface is covered by the otolithic membrane (statoconial membrane), a gelatinous structure in which many small crystals, the otoconia (otoliths, statoliths), are embedded. A curved ridge, the ‘snowdrift line’, runs along the length of the otolithic membrane. It corresponds to a narrow crescent of underlying sensory epithelium termed the striola, 0.13 μm wide. The density of sensory hair cells in this strip of epithelium is 20% less than in the rest of the macula. The striola is convex laterally and runs from the medial aspect of the anterior margin in a posterior direction towards, but not reaching, the posterior pole. The part of the macula medial to the striola is called the pars interna and is slightly larger than the pars externa, which is lateral to it. The significance of this area is that the sensory cells are functionally and anatomically polarized towards it (Fig. 37.6). The macula in each utricle is approximately horizontal when the head is in its normal position. Linear acceleration of the head in any horizontal plane will result in the otolithic membrane lagging behind the movement of the membranous labyrinth as a result of the inertia produced by its mass. The membrane thus maximally stimulates one group of hair cells by deflecting their bundles towards the striola whilst inhibiting others by deflecting their bundles away from it. Hence each horizontal movement of the head will produce a specific pattern of firing in the utricular efferents.
Saccule
The saccule (or sacculus) is a slightly elongated, globular sac lying in the spherical recess near the opening of the scala vestibuli of the cochlea (Fig. 37.6). As already noted, it is connected to the utricle and endolymphatic duct by the utriculosaccular duct, and to the cochlea by the ductus reuniens, which leaves inferiorly to open into the base of the cochlear duct (Fig. 37.3A).
The saccular macula is an almost elliptical structure, 2.6 mm long and 1.2 mm at its widest point. Its long axis is orientated anteroposteriorly but, in contrast to the utricular macula, the saccular macula lies in a vertical plane on the wall of the saccule. Its elliptical shape is very slightly distorted by a small anterosuperior bulge. Like the utricular macula, it is covered by an otolithic (statoconial) membrane and possesses a striola, 0.13 mm wide, which extends along its long axis as an S-shaped strip about which the sensory cells are functionally and anatomically polarized (Fig. 37.6). The part of the macula above the striola is termed the pars interna, and that below it, the pars externa. The operation of the saccule is similar to that of the utricle. However, because of its vertical orientation, the saccule is particularly sensitive to linear acceleration of the head in the vertical plane, and is, therefore, a major gravitational sensor when the head is in an upright position. It is also particularly sensitive to movement along the anteroposterior axis.
Semicircular canals
The lateral, anterior and posterior semicircular ducts follow the course of their osseous canals. Throughout most of their length they are securely attached, by much of their circumference, to the osseous walls. They are approximately one-quarter of the diameter of their osseous canals (Fig. 37.3B). The medial ends of the anterior and posterior canals fuse to form a single common duct, the crus commune, before entering the utricle. The lateral end of each canal is dilated to form an ampulla, which lies within the ampulla of the osseous canal. The short segment of duct between the ampullae and utricle is the crus ampullaris.
The membranous wall of each ampulla contains a transverse elevation (septum transversum) on the central region of which is a sensory area, the ampullary crest (crista). This is a saddle-shaped ridge that lies transversely across the duct. It is broadly concave on its free edge along most of its length and has a concave gutter (planum semilunatum) at either end between the ridge and the duct wall. Sectioned across the ridge, the crests of the lateral and anterior semicircular canals have smoothly rounded corners; the posterior crest is more angular. A vertical plate of gelatinous extracellular material, the cupula, is attached along the free edge of the crest (Fig. 37.7). It projects far into the lumen of the ampulla so that movements of endolymph within the duct readily deflect the cupula and therefore also the stereocilia of the sensory cells that are inserted into its base. The three semicircular canals thus detect angular accelerations during tilting or turning movements of the head in any direction.
Microstructure of the vestibular system
Type I vestibular sensory cells measure 25 μm in length, with a free surface of 6–7 μm in diameter. The basal part of the cell does not reach the basal lamina of the epithelium. Each cell is typically bottle-shaped, with a narrow neck and a rather broad, rounded basal portion containing the nucleus (Fig. 37.6). The apical surface is characterized by 30–50 stereocilia (large, regularly-arranged, modified microvilli about 0.25 μm across) and a single kinocilium (with the typical ‘9 + 2′ arrangement of microtubules characteristic of true cilia). The kinocilium is considerably longer than the stereocilia, and may attain 40 μm, whereas the stereocilia are of graded lengths. They are characteristically arranged in regular rows behind the kinocilium in descending order of height, the longest being next to the kinocilium (Fig. 37.8). The kinocilium emerges basally from a typical basal body, with a centriole immediately beneath it. Close to the inner surface of their basal two-thirds, every cell contains numerous synaptic ribbons with associated synaptic vesicles (see p. 45). The postsynaptic surface of an afferent nerve ending encloses the greater part of the sensory cell body in the form of a cup (chalice or calyx). Efferent nerve fibres make synapses with the external surface of the calyx, rather than directly with the sensory cell.
There is much greater variation in the sizes of type II sensory cells (Fig. 37.9). Some are up to 45 μm long, and almost span the entire thickness of the sensory epithelium, whereas others are shorter than type I cells. They are mostly cylindrical, but otherwise resemble type I cells in their contents and the presence of an apical kinocilium and stereocilia. However, their kinocilia and stereocilia tend to be shorter and less variable in length. The most striking difference between type I and II cells is their efferent nerve terminals: type II cells receive several efferent nerve boutons containing a mixture of small clear and dense-core vesicles around their bases. Afferent endings are small expansions rather than chalices.
Each sensory cell is structurally and functionally polarized (Fig. 37.6). Deflection of the hair bundle towards the kinocilium results in depolarization of the hair cell, and increases the rate of neurotransmitter release from its base. Deflection away from the kinocilium hyperpolarizes the hair cell and reduces the release of neurotransmitter. The hair cells have specific orientations within each sensory organ. In the maculae, they are arranged symmetrically on either side of the striola. In the utricle, the kinocilia are positioned on the side of the sensory cell nearest to the striola. In the saccule, they are furthest from it. In the ampullary crests, the cells are orientated with their rows of stereocilia at right angles to the long axis of the semicircular duct. In the lateral crest the kinocilia are on the side towards the utricle, whereas in the anterior and posterior crests they are away from it. These different arrangements are important functionally, because any given acceleration of the head maximally depolarizes one group of hair cells and maximally inhibits a complementary set, thus providing a unique representation of the magnitude and orientation of any movement (for further details, see Furness 2002).
The type I and II sensory cells are set within a matrix of supporting cells that reach from the base of the epithelium to its surface, and form rosettes round the sensory cells, as seen in surface view. Although their form is irregular, they can easily be recognized by the position of their nuclei, which tend to lie below the level of sensory cell nuclei and just above the basal lamina (Fig. 37.5). The apices of the supporting cells are attached by tight junctions to neighbouring cells to produce the reticular lamina, a composite layer which forms a plate that is relatively impermeable to cations other than via the mechanosensitive transduction channels of the hair cells.
The otolithic membrane is a layer of extracellular material with a complex structure. It can be divided into two strata. The external layer is composed of otoliths or otoconia, which are barrel-shaped crystals of calcium carbonate with angular ends, up to 30 μm long, and heterogeneous in distribution (Fig. 37.6). They are attached to a more basal gelatinous layer into which the stereocilia and kinocilia of the sensory cells are inserted (Fig. 37.8). The gelatinous material consists largely of glycosaminoglycans associated with fibrous protein.
Endolymphatic duct and sac
The endolymphatic duct runs in the osseous vestibular aqueduct and becomes dilated distally to form the endolymphatic sac. This is a structure of variable size, which may extend through an aperture on the posterior surface of the petrous bone to end between the two layers of the dura on the posterior surface of the petrous temporal bone near the sigmoid sinus (Fig. 37.3A). The surface cells throughout the entire endolymphatic duct resemble those lining the non-specialized parts of the membranous labyrinth and consist of squamous or low cuboidal epithelium. The epithelial lining and subepithelial connective tissue become more complex where the duct dilates to form the endolymphatic sac. An intermediate or rugose segment and a distal sac can be distinguished. In the intermediate segment, the epithelium consists of light and dark cylindrical cells. Light cells are regular in form and have numerous long surface microvilli with endocytic invaginations between them and large clear vesicles in their apical region. In contrast, dark cells are wedge-shaped and have a narrow base, few apical microvilli and dense, fibrillar cytoplasm.
COCHLEAR DUCT
The cochlear duct is a spiral tube that runs within the bony cochlea (Figs 37.2A and B, 37.3). The osseous spiral lamina projects for part of the distance between the modiolus and the outer wall of the cochlea and is attached to the inner edge of the basilar membrane. The endosteum of the outer wall is thickened to form a spiral cochlear ligament which projects inwards as a triangular basal crest attached to the outer rim of the basilar membrane. Immediately above this is a concavity, the external spiral sulcus (sulcus spiralis externus), above which the thick, highly vascular periosteum projects as a spiral prominence. Above the prominence is a specialized, thick epithelial layer, the stria vascularis. A second, thinner vestibular membrane, Reissner’s membrane, extends from the thickened endosteum on the osseous spiral lamina to the outer wall of the cochlea, where it is attached above the stria. Reissner’s membrane consists of two layers of squamous epithelial cells separated by a basal lamina. The side facing the scala vestibuli bears flattened perilymphatic cells, with tight junctions between them, creating a diffusion barrier. The endolymphatic side is lined by squamous epithelial cells; these are also joined by tight junctions and are involved in ion transport. The canal thus enclosed between the scala tympani and the scala vestibuli is the cochlear duct (Fig. 37.2B). It is triangular in cross-section throughout the length of the cochlea. Its closed upper end, the lagena, is attached to the cupula. The lower end of the duct turns medially, narrowing into the ductus reuniens, and connects with the saccule (Fig. 37.3A).
The stria vascularis lies on the outer wall of the cochlear duct, above the spiral eminence (Fig. 37.2B and C). It has a special stratified epithelium containing a dense intraepithelial capillary plexus and three cell types: superficial marginal, dark or chromophil cells; intermediate light, or chromophobe cells, and basal cells. The endolymphatic surface consists only of the apices of marginal cells. The intermediate and basal cells lie deeper within the stria and send cytoplasmic processes towards the surface, between the deeper parts of the marginal cells. The long descending cytoplasmic processes of the marginal dark cells and the ascending processes of the intermediate and basal cells envelop the intraepithelial capillaries. The stria vascularis is involved in ion transport, and it helps to produce the unusual ionic composition of endolymph. It is the source of the large positive endocochlear electrical potential, maintenance of which is directly dependent upon adequate oxygenation of the epithelial cells which is provided by the intraepithelial capillary plexus.
The osseous spiral lamina consists of two plates of bone between which are canals for the cochlear nerve filaments. On the upper plate, the periosteum is thickened to form the spiral limbus (limbus laminae spiralis) (Fig. 37.2B). It ends externally in the internal spiral sulcus, which in section is shaped like a C. Its upper part, the overhanging limbic edge, is the vestibular labium and the lower tapering part is the tympanic labium which is perforated by small holes (the habenula perforata) for branches of the cochlear nerve. The upper surface of the vestibular labium is crossed at right angles by furrows, separated by numerous elevations, the auditory teeth (dentes acustici). The limbus is covered by a layer that appears superficially to be squamous epithelium, however, only the cells over the ‘teeth’ are flat, and those in the furrows are flask-shaped interdental cells. The epithelium is continuous with the epithelium in the internal spiral sulcus and on the inferior surface of Reissner’s membrane. During development the interdental cells secrete some of the material that forms the tectorial membrane.
Basilar membrane
The basilar membrane stretches from the tympanic lip of the osseous spiral lamina to the basal crest of the spiral ligament (Fig. 37.2B and C). It consists of two zones. The thin zona arcuata stretches from the spiral limbus to the bases of the outer pillar cells and supports the organ of Corti. It is composed of compact bundles of small (8–10 nm diameter) collagenous filaments, mainly radial in orientation. The outer thicker zona pectinata starts beneath the bases of the outer pillar cells and is attached to the crista basilaris. The basilar membrane is trilaminar in the zona pectinata, but the upper and lower layers fuse at its attachment to the crista basilaris. The length of the basilar membrane is 35 mm; its width increases from 0.21 mm basally to 0.36 mm at its apex, accompanied by corresponding narrowing of the osseous spiral lamina and a decrease in the thickness of the basal crest. The lower or tympanic surface of the basilar membrane is covered by a layer of vascular connective tissue and elongated perilymphatic cells. One vessel, the spiral vessel (vas spirale), is larger; it lies immediately below the tunnel of Corti.
Organ of Corti
The organ of Corti consists of a series of epithelial structures that lie on the zona arcuata of the basilar membrane (Fig. 37.2B and C). The more central of these structures are two rows of cells, the internal and external pillar cells. The bases of the pillar cells are expanded, and rest contiguously on the basilar membrane, but their rod-like cell bodies are widely separated. The two rows incline towards each other and come into contact again at the heads of the pillars, enclosing between them and the basilar membrane the tunnel of Corti, which has a triangular cross-section (Fig. 37.2C). Internal to the inner pillar cells is a single row of inner hair cells. External to the outer pillar cells are three or four rows of outer hair cells. The bases of the outer hair cells are cupped by supporting cells called outer phalangeal (Deiters’) cells, except for a gap where cochlear axons synapse with them. The apical ends of the hair cells and apical processes of the supporting cells form a regular mosaic called the reticular lamina, which is covered by the tectorial membrane, a gel-like structure projecting from the spiral limbus. The reticular lamina is impervious to ions and thus maintains the electrochemical gradient between the fluids surrounding the apices and the basolateral membranes of the sensory hair cells. A narrow gap separates the tectorial membrane from the reticular lamina except where the apical stereocilia of the outer hair cells project to make contact with it.
Each pillar cell has a base or crus, an elongated scapus (rod) and an upper end or caput (head) (Fig. 37.2C); each crus and caput are in contact, but the scapi are separated by the tunnel of Corti. Electron microscopy shows many microtubules, 30 nm in diameter, arranged in linked parallel bundles of 2000 or more in the scapus, originating in the crus and diverging above the scapus to terminate in the head region. The nucleus is situated in the foot-like expansion resting on the basal lamina.
Cochlear hair cells are the sensory transducers of the cochlea: collectively, they detect the amplitude and frequency of the sound waves that enter the cochlea. All cochlear hair cells have a common pattern of organization. They are elongated cells with a group of modified apical microvilli or stereocilia (which contain parallel arrays of actin filaments) and a group of synaptic contacts with cochlear axons at their rounded bases (Fig. 37.10B). The inner hair cells form a single row along the inner edge of inner pillar cells (and the spiral tunnel), whereas the outer hair cells are arranged in three or, in some regions of the human cochlea, in four or even five rows, interspersed with supporting cells (Fig. 37.10A). These two groups have distinctive roles in sound reception; the differences in their detailed structure reflect this functional divergence. There are 3500 inner hair cells and 12,000 outer hair cells. The two sets of hair cells lean towards each other apically at about the same angles as the neighbouring inner and outer pillar cells. The geometric arrangement of these cells is very precise, and this pattern is closely related to the sensory performance of the cochlea.
The inner hair cells are pear-shaped and slightly curved; the narrower end is directed towards the surface of the organ of Corti and the wider basal end is positioned some distance above the inner end of the basilar membrane (Fig. 37.2C). The inner hair cells are surrounded by inner border cells and by inner phalangeal cells which are attached externally to the heads of the inner pillar cells. The flat apical surface of each inner hair cell is elliptical when viewed from above, its long axis directed in the direction of the row of hair cells (Fig. 37.10A). The breadth of the apex exceeds that of the inner pillar cells so that each inner hair cell is related to more than one inner pillar cell. The apex bears 50–60 stereocilia, arranged in several ranks of progressively ascending height, the tallest on the strial side. The tips of the shorter rows are connected diagonally to the sides of the adjacent taller stereocilia by thin filaments called tip links; each stereocilium is also connected to all its neighbours by lateral links. The length of a stereociliary row varies along the length of the cochlea, being longest at the apex and shortest at its base. The stereociliary bases insert into a transverse lamina of dense fibrillar material, the cuticular plate, which lies immediately beneath the apical surface of each inner hair cell. The cuticular plate includes a small aperture containing a basal body. During development, a kinocilium containing microtubules is anchored here, a condition which persists in vestibular hair cells.
Outer hair cells are long cylindrical cells which are nearly twice as tall as the inner hair cells (Figs 37.2C, 37.6, 37.10A). There is a gradation of length: the outermost row is longest in any one cochlear region, and those of the cochlear apex are taller than those of the base. They are surrounded by the apical or phalangeal processes of the Deiters’ cells or, on the internal side of the inner row, by the heads of the outer pillar cells. The stereocilia, which may number up to 100 per cell, are arranged in three rows of graded heights; the longest is on the outer side. The rows are arranged in the form of a V or W depending on cochlear region, the points of the angles directed externally. The stereocilia are also graded in length according to cochlear region: those of the cochlear base are shortest. Like those of inner hair cells, the stereocilia possess tip links and other filamentous connections with their neighbours, and are inserted at their narrow bases into a cuticular plate. The tallest stereocilia are embedded in shallow impressions on the underside of the tectorial membrane.
Cochlear hair cells respond with phenomenal speed and sensitivity to sound vibrations that cause submicron deflections of their stereociliary hair bundles. Outer hair cells not only detect these vibrations but also generate force to increase auditory sensitivity and frequency discrimination. (See Fettiplace & Hackney 2006, for further details about the sensory and motor functions of auditory hair cells.)
Deiters’ or phalangeal cells lie between the rows of outer hair cells. Their expanded bases lie on the basilar membrane and their apical ends partially envelop the bases of the outer hair cells (Figs 37.2C and 37.11). Each has a finger-like (phalangeal) process that extends diagonally upwards between the hair cells to the reticular membrane, where it forms a plate-like expansion that fills the gaps between hair cell apices.
Five or six rows of columnar supporting cells or external limiting cells, such as Hensen’s cells and Claudius’ cells lie external to the Deiters’ cells (Fig. 37.2C).
Tectorial membrane
The tectorial membrane overlies the sulcus spiralis internus and organ of Corti and is a stiff, gelatinous plate (Fig. 37.2B and C). It contains collagen types II, V and IX, interspersed with glycoproteins (tectorins), which contribute approximately half of the total protein.
In transverse section, the tectorial membrane has a characteristic shape. The underside is nearly flat and the upper surface is convex, and it is thin on the modiolar side where it is attached to the vestibular labium of the spiral limbus. Its outer part forms a thickened ridge, overhanging the edge of the reticular lamina. The lower surface is relatively smooth, except where the stereocilia of the outer hair cells are embedded in the membrane, leaving a pattern of W- or V-shaped impressions, an S-shaped ridge called Hensen’s stripe which projects towards the stereocilia of the inner hair cells. The interdental cells of the spiral limbus are believed to secrete the membrane.
VASCULAR SUPPLY
INNERVATION
VESTIBULOCOCHLEAR NERVE
The vestibulocochlear nerve emerges from the cerebellopontine angle (see Fig. 19.3). It courses through the posterior cranial fossa to enter the petrous temporal bone via the internal acoustic meatus, where it divides into an anterior trunk, the cochlear nerve, and a posterior trunk, the vestibular nerve (Fig. 37.12A; see Fig. 27.8). Both contain the centrally directed axons of bipolar neurones, together with a smaller number of efferent fibres that arise from brain stem neurones and terminate on cochlear and vestibular sensory cells. In humans, the intratemporal portion of the vestibulocochlear nerve consists of two histologically distinct portions: a central glial zone adjacent to the brain stem, and a peripheral or non-glial zone (Bridger & Farkashidy 1980). In the glial zone the axons are supported by central neuroglia, whereas in the non-glial zone they are ensheathed by Schwann cells. The non-glial zone extends into the cerebellopontine angle medial to the internal acoustic meatus in more than 50% of human vestibulocochlear nerves. During development, a gap of several weeks has been reported between the onset of Schwann cell myelination distally and glial myelination proximally: it has been suggested that the gap may coincide with the time of the final maturation of the organ of Corti. (For further details about the development of the human cochlear nerve see Ray et al 2005.)
Vestibular nerve
The cell bodies of the bipolar neurones that contribute to the vestibular nerve lie in the vestibular ganglion, which is situated in the trunk of the nerve within the lateral end of the internal auditory meatus (Fig. 37.12B). Their peripheral processes innervate the maculae of the utricle and saccule and the ampullary crests of the semicircular canals (see below). Their axons travel to the CNS in the vestibular nerve, which enters the brain stem at the cerebellopontine angle, and terminates in the vestibular nuclear complex. Neurones in this complex project to motor nuclei in the brain stem and upper spinal cord, and to the cerebellum and thalamus. Thalamic efferent projections pass to a cortical vestibular area which is probably located near the intraparietal sulcus in area 2 of the primary somatosensory cortex.
Vestibular (Scarpa’s) ganglion
The cell bodies of the neurones in the vestibular ganglion vary considerably in size: their circumferences range from 45 to 160 μm (Felix et al 1987). No topographically ordered distribution relating to size has been found. The cell bodies are notable for their abundant granular endoplasmic reticulum, which in places forms Nissl bodies, and prominent Golgi complexes. They are covered by a thin layer of satellite cells and are often arranged in pairs, closely abutting each other so that only a thin layer of endoneurium separates the adjacent coverings of satellite cells. This arrangement has led to speculation that ganglion cells may affect each other directly by electrotonic spread (ephaptic transmission: see Felix et al 1987).
Intratemporal vestibular nerve
The peripheral processes of the vestibular ganglion cells are aggregated into definable nerves, each with a specific distribution. The main nerve divides at and within the ganglion into superior and inferior divisions, which are connected by an isthmus. The superior division, the larger of the two, passes through the small holes in the superior vestibular area at the fundus of the internal acoustic meatus (Fig. 37.4) and supplies the ampullary crests of the lateral and anterior semicircular canals via the lateral and anterior ampullary nerves, respectively. A secondary branch of the lateral ampullary nerve supplies the macula of the utricle; however, the greater part of the utricular macula is innervated by the utricular nerve, which is a separate branch of the superior division. Another branch of the superior division, Voit’s nerve, supplies part of the saccule.
The inferior division of the vestibular nerve passes through small holes in the inferior vestibular area (Fig. 37.4) to supply the remainder of the saccule and the posterior ampullary crest via saccular and singular branches, respectively; the latter passes through the foramen singulare. Occasionally, a very small supplementary or accessory branch innervates the posterior crest; it is probably a vestigial remnant of the crista neglecta, an additional area of sensory epithelium found in some other mammals but seldom in man.
Afferent and efferent cochlear fibres are also present in the inferior division of the vestibular nerve, but leave at the anastomosis of Oort to join the main cochlear nerve (see review by Warr 1992). Another anastomosis, the vestibulofacial anastomosis, is situated more centrally between the facial and vestibular nerves, and is the point at which fibres originating in the intermediate nerve pass from the vestibular nerve to the main trunk of the facial nerve.
Anatomy of balance and posture
The stereocilia in the apical hair bundles of the mechanosensitive hair cells in each of these organs are embedded in an overlying accessory gel-like structure, the otolithic membrane (in the utricle and the saccule) and the cupula (in the semicircular canals). Their apical surfaces are bathed in endolymph: tight junctional complexes between the apices of the hair cells and their adjacent supporting cells separate the endolymph from the perilymph that bathes their basolateral surfaces. Deflection of the stereocilia (caused by displacements of their overlying accessory membranes by fluid movements in the membranous labyrinth) produces either an increased or decreased rate of opening of the mechanotransduction channels at their tips, depending on whether they are deflected towards or away from the tallest row respectively. The change in the membrane potential of the receptor cell is signalled to the brain as a change in the firing frequency of the vestibular nerve afferents (either an increase or a decrease of the basal resting discharge, depending on the direction of stimulation). The signals are compared centrally with visual and somatosensory signals, which also signal the position of the head in space (for a more detailed account, see Furness 2002).
Semicircular canals
Angular acceleration and deceleration of the head cause a counterflow of endolymph in the semicircular canals, which deflects the cupola of each crista and bends the stereociliary/kinociliary bundles. When a steady velocity of head movement is reached, the endolymph rapidly adopts the same velocity as the surrounding structures because of friction with the canal walls, so that the cupula and receptor cells return to their resting state. The three semicircular canals are orientated at right angles to each other, which means that all possible directions of acceleration can be detected. Directional sensitivity to head movement is coded by opposing receptor signals: the left and right semicircular canals of each functional pair (e.g. the left and right superior canals), respond oppositely to any movement of the head that affects them (Fig. 37.13). Some vestibular neurones receive a bilateral input from vestibular receptors, which means that they can compare the discharge rates of right and left canal afferents, a mechanism that increases the sensitivity of the system.
Maculae
In the maculae, the weight of the otoconial crystals creates a gravitational pull on the otoconial membrane and thus on the stereociliary bundles of the sensory cells which are inserted into its base. Because of this, they are able to detect the static orientation of the head with respect to gravity. They also detect shifts in position according to the extent to which the stereocilia are deflected from the perpendicular. The two maculae are set at right angles to each other, and the cells of both maculae are orientated functionally in opposite directions across their striolar boundaries. Movement causes depolarization of the hair cells on one side of the striola and hyperpolarization of cells on the other side; because the striola is curved, small groups of hair cells on the macular epithelium each respond to a specific direction of head tilt or linear acceleration (Fig. 37.14). Moreover, because the otoconia have a collective inertia/momentum, linear acceleration and deceleration along the anteroposterior axis can be detected by the lag or overshoot of the otoconial membrane with respect to the epithelial surface, and so the saccular macula is able to signal these changes of velocity.
Visual reflexes
The vestibular system plays a major role in the control of visual reflexes, which allow the fixation of gaze on an object in spite of movements of the head, and require the coordinated movements of the eye, neck and upper trunk. Constant adjustments of the visual axes are achieved chiefly through the medial longitudinal fasciculus, which connects the vestibular nuclear complex with neurones in the oculomotor, trochlear and abducens nuclei and with upper spinal motor neurones (Fig. 37.15; see Fig. 39.12), and also by the vestibulospinal tracts.
Cochlear nerve
Intratemporal cochlear nerve
The cochlear nerve connects the organ of Corti to the cochlear and related nuclei of the brain stem. The cochlear nerve lies inferior to the facial nerve throughout the internal acoustic meatus (see above). It becomes intimately associated with the superior and inferior divisions of the vestibular nerve, which are situated in the posterior compartment of the canal, and leaves the internal acoustic meatus in a common fascicle (Fig. 37.12A).
There are approximately 30–40,000 nerve fibres in the human cochlear nerve (for review, see Nadol 1988). Their fibre diameter distribution is unimodal, and ranges from 1 to 11 μm, with a peak at 4–5 μm. Functionally, the nerve contains both afferent and efferent somatic fibres, together with adrenergic postganglionic sympathetic fibres from the cervical sympathetic system.
Afferent cochlear innervation
The afferent fibres are myelinated axons with bipolar cell bodies that lie in the spiral ganglion in the modiolus (Fig. 37.2B; Fig. 37.16). There are two types of ganglion cell: most (90–95%) are large type I cells, the remainder are smaller type II cells (see reviews by Nadol 1988, Eybalin 1993). Type I cells contain a prominent spherical nucleus, abundant ribosomes and many mitochondria; in many mammals (although possibly not in humans) they are surrounded by myelin sheaths. In contrast, type II cells are smaller, always unmyelinated, and have a lobulated nucleus. The cytoplasm of type II cells is enriched with neurofilaments, but has fewer mitochondria and ribosomes than type I cells.
Three distinct groupings of afferent fibres have been identified: inner radial, basilar and outer spiral fibres (Fig. 37.17).
Efferent cochlear fibres
The efferent nerve fibres in the cochlear nerve are derived from the olivocochlear system (see reviews by Warr 1992, Guinan 1996). Within the modiolus, the efferent fibres form the intraganglionic spiral bundle, which may be one or more discrete groups of fibres situated at the periphery of the spiral ganglion (Fig. 37.17). There are two main groups of olivocochlear efferents: lateral and medial. The lateral efferents come from small neurones in and near the lateral superior olivary nucleus and arise mainly, but not exclusively, ipsilaterally. They are organized into inner spiral fibres that run in the inner spiral bundle before terminating on the afferent axons that supply the inner hair cells. The medial efferents originate from larger neurones in the vicinity of the medial superior olivary nucleus, and the majority arise contralaterally. They are myelinated and cross the tunnel of Corti to synapse with the outer hair cells mainly by direct contact with their bases, although a few synapse with the afferent terminals. The efferent innervation of the outer hair cells decreases along the organ of Corti from cochlear base to apex, and from the first (inner) row to the third. The efferents use acetylcholine, γ-aminobutyric acid (GABA), or both as their neurotransmitter. They may also contain other neurotransmitters and neuromodulators.
Activity of the medial efferents inhibits cochlear responses to sound: the strength of the activity grows slowly with increasing sound level. They are believed to modulate the micromechanics of the cochlea by altering the mechanical responses of the outer hair cells thus changing their contribution to frequency selectivity and sensitivity. The lateral efferents related to the inner hair cells also respond to sound. They appear to modify transmission through their postsynaptic action on inner hair cell afferents. The cholinergic fibres may excite the radial fibres, while those containing GABA may inhibit them, although their role is less well understood than that of the medial efferents (see review by Guinan 1996).
Anatomy of hearing
Sounds waves entering the external ear are converted into electrical signals in the cochlear nerve by the peripheral auditory system (Fig. 37.18). The axons in the cochlear nerve constitute the auditory component of the vestibulocochlear nerve and terminate in the dorsal and ventral cochlear nuclei: onward connections make up the ascending (central) auditory pathway.
Peripheral auditory system
Vibrations in the air column in the external acoustic meatus cause a comparable set of vibrations in the tympanic membrane and auditory ossicles. The chain of ossicles acts as a lever which increases the force per unit area at the round window by 1.2 times while the reduction in size of the round window compared with the tympanic membrane increases the force per unit area of the oscillating surface a further 17 times. This overcomes the inertia of the cochlear fluids and produces in them pressure waves that are conducted almost instantaneously to all parts of the basilar membrane. The latter varies continuously in width, mass and stiffness from the basal to the apical end of the cochlea. Each part of the basilar membrane vibrates, but only the region tuned to a specific frequency will respond maximally to a pure tone entering the ear. A wave of mechanical motion, the travelling wave, is propagated along the basilar membrane to the position where it responds maximally and then dies away again. With increasing frequency, the locus of maximum amplitude moves progressively from the apical to the basal end of the cochlea. The pattern of vibrations in the basilar membrane thus varies with the intensity and frequency of the acoustic waves reaching the perilymph. Because of the arrangement of the hair cells on the basilar membrane, these oscillations generate a largely transverse shearing force between the outer hair cells and the overlying tectorial membrane (in which the apices of the hair cell stereocilia are embedded). This movement depends on the mechanical properties of the entire organ of Corti, including its cytoskeleton, which stiffens this structure. The inner hair cell stereocilia, which probably do not touch the tectorial membrane although they come very close to it, are likely to be stimulated by local movements of the endolymph. Displacement of the stereociliary bundle of a hair cell activates mechanoelectrical transduction (MET) ion channels near the tips of its stereocilia, and this allows potassium and calcium ions from the endolymph to enter the hair cell (see overviews by Fettiplace 2002, Fettiplace & Hackney 2006). This induces a depolarizing receptor potential and the release of neurotransmitter onto the cochlear afferents at the base of the cell. In this way a specific group of auditory axons is activated at the position of maximal basilar membrane vibration.
The mechanical behaviour of the basilar membrane is responsible for a rather broad discrimination between different frequencies (passive tuning, see overview by Ashmore 2002), but fine frequency discrimination in the cochlea appears to be related to physiological differences between the hair cells. Individual tuning of hair cells may result from differences in shape, stereociliary length, or possibly variations in the molecular composition of sensory membranes, and may have a role in cochlear amplification (active tuning).
The activity of the outer hair cells appears to play an important part in regulating inner hair cell sensitivity at specific frequencies. Outer hair cells can change length when stimulated electrically at frequencies of many thousands of cycles per second. The rapidity of these changes in length indicates a novel type of motile mechanism, which is believed to depend on conformational changes in proteins located in the plasma membrane of the cells (see Fettiplace & Hackney 2006) (Fig. 37.19). When the membrane potential of the outer hair cells changes, they generate forces along their axes. When the mechanoelectrical transduction channels open, they are thought to oppose the viscous forces which tend to damp down the vibration of the cochlear partition, and adjust the mechanics of the organ of Corti on a cycle-by-cycle basis. Alternatively they may alter the mechanics of the partition more slowly under the influence of the efferent pathway.
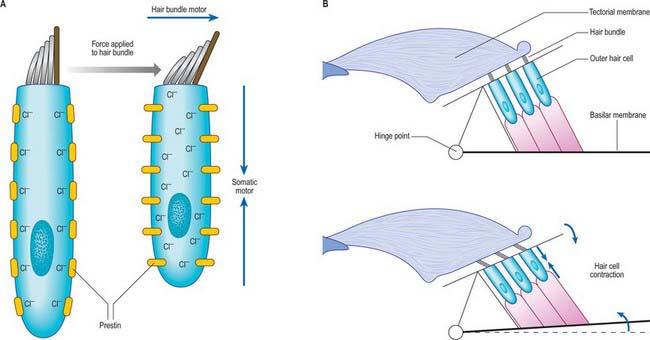
Fig. 37.19 The putative motors of outer hair cells. Outer hair cells can generate force, mechanically boosting sound-induced vibrations of the hair bundle and augmenting frequency tuning. Two mechanisms have been advanced to explain this cochlear amplifier: the somatic motor and the hair bundle motor. A, In the resting state, Cl− ions are bound to prestin molecules in the lateral membrane of the hair cell. When force is applied to the hair bundle, the cell is depolarized, the Cl− ions dissociate and the prestin changes conformation, reducing its area in the plane of the membrane and shortening the hair cell body (the somatic motor). Adaptation of mechanoelectrical transduction (MET) channels, which are activated by bending of the stereocilia at their tapered base, also causes the hair bundle to produce extra force in the direction of the stimulus (the hair bundle motor). The amplitudes of the hair bundle movements have been exaggerated to illustrate the concept. B, The effects of the somatic motor (blue arrows) on the organ of Corti mechanics, which leads to downward motion of the reticular lamina (the upper surface of the organ of Corti) and a negative deflection of the hair bundle. This is a negative feedback pathway, as a positive deflection of the hair bundle causes outer hair cell depolarization, cell contraction and opposing motion of the bundle. (See Fettiplace & Hackney 2006.)
At a particular frequency, an increase in the intensity of stimulus is signalled by an increase in the rate of discharge in individual cochlear axons. At greater intensities it is signalled by the number of activated cochlear axons (recruitment).
Under stimulation by sound, a rapid oscillatory cochlear microphonic potential can be recorded. It matches the frequency of the stimulus and movements of the basilar membrane precisely, and appears to depend on fluctuations in the conductance of hair cell membranes, probably of the outer hair cells. At the same time, an extracellular summating potential develops, a steady direct current shift related to the (intracellular) receptor potentials of the hair cells. Cochlear nerve fibres then begin to respond with action potentials which are also recordable from the cochlea. Intracellular recording of auditory responses from inner hair cells has confirmed that these cells resemble other receptors: their steady receptor potentials are related in size to the amplitude of the acoustic stimulus. At the same time, afferent axons are stimulated by synaptic action at the bases of the inner hair cells. They fire more rapidly as the vibration of the basilar membrane increases in amplitude, up to a threshold that depends on the sensitivity of the specific nerve fibre involved. Each inner hair cell is contacted by axons with response thresholds that range from 0 decibels sound pressure level (dBSPL), the approximate threshold of human hearing, to those which respond to intensities in excess of 100 dBSPL; the loudest sound tolerable is around 120 dBSPL. Each axon responds most sensitively to the frequency represented by its particular cochlear location, its characteristic frequency (Fig. 37.18).
Central auditory pathway
The primary afferents of the auditory pathway arise from cell bodies in the spiral ganglion of the cochlea. The axons travel in the vestibulocochlear nerve, which enters the brain stem at the cerebellopontine angle. Afferent fibres bifurcate, and terminate in the dorsal and ventral cochlear nuclei (Fig. 37.20). The dorsal cochlear nucleus projects via the dorsal acoustic stria to the contralateral inferior colliculus. The ventral cochlear nucleus projects via the trapezoid body or the intermediate acoustic stria to relay centres in either the superior olivary complex, the nuclei of the lateral lemniscus, or the inferior colliculus. The superior olivary complex is dominated by the medial superior olivary nucleus which receives direct input from the ventral cochlear nucleus on both sides, and is involved in localization of sound by measuring the time difference between afferent impulses arriving from the two ears.
The inferior colliculus consists of a central nucleus and two cortical areas. The dorsal cortex lies dorsomedially, and the external cortex lies ventromedially. Secondary and tertiary fibres ascend in the lateral lemniscus. They converge in the central nucleus, which projects to the ventral division of the medial geniculate body of the thalamus. The external cortex receives both auditory and somatosensory input. It projects to the medial division of the medial geniculate body, and, together with the central nucleus, also projects to olivocochlear cells in the superior olivary complex and to cells in the cochlear nuclei. The dorsal cortex receives an input from the auditory cortex and projects to the dorsal division of the medial geniculate body (see Ch. 21). Connections also run from the nucleus of the lateral lemniscus to the deep part of the superior colliculus, to coordinate auditory and visual responses.
The ascending auditory pathway crosses the midline at several points both below and at the level of the inferior colliculus. However, the input to the central nucleus of the inferior colliculus and higher centres has a clear contralateral dominance: during the initial stages of cortical auditory processing, both hemispheres respond most strongly to the contralateral ear. The medial geniculate body is connected reciprocally to the primary auditory cortex, which lies in the posterior half of the superior temporal gyrus and also dives into the lateral sulcus as the transverse temporal gyri (Heschl’s gyri) (see Ch. 23). Secondary areas of the auditory cortex are located in an adjacent belt region, and other regions of auditory association cortex have been described in a parabelt region beyond the secondary cortex.
The corpus callosum, particularly the posterior third of the body, contains auditory interhemispheric fibres that originate from the primary and second auditory cortices. Asymmetries of minicolumn number in primary and association auditory regions have been correlated with axonal fibre numbers in the subregions of the corpus callosum through which they project (Chance et al 2006).
The transformation of the physical characteristics of sound into ‘auditory objects’ is thought to occur in the transition from primary to secondary auditory cortex. (For a critical perspective on auditory objects, see Griffiths & Warren 2004.)
Deafness
Conductive hearing loss may result from trauma to the external or middle ears, blockage of the external auditory meatus, or disruption of the tympanic membrane (e.g. by intense sounds or extreme pressure changes) (Ch. 36). It may also result from chronic inflammation of the tympanic membrane (e.g. by a cholesteatoma, which may also damage the ossicles); from an infection of the middle ear (otitis media with effusion), which produces a fluid build-up in the normally air-filled middle ear and so impedes the movements of the ossicles; or from otosclerosis, an inappropriate thickening of bone around the footplate of the stapes.
Sensorineural hearing loss is the most prevalent form of hearing impairment. It refers to loss or damage of the sensory hair cells or their innervation. The sensory cells of the inner ear are particularly vulnerable to mechanical trauma produced by high intensity noise and to changes in their physiological environment caused by infection or hypoxia. Changes in their ionic environment rapidly lead to degenerative processes that result in hair cell loss, often by apoptosis, and produce either hearing loss or vestibular dysfunction. These changes can be induced by drugs such as the aminoglycoside antibiotics, some diuretics, and certain anticancer drugs. A decrease in cochlear sensitivity, presbyacusis, almost invariably occurs with age: hair cells at the high frequency end of the cochlea tend to be lost first. At least 60% of hearing loss may have a genetic basis, a significant proportion may be non-syndromic, and most of these genes are inherited in an autosomal recessive mode.
Ashmore J. The mechanics of hearing. In: Roberts D, editor. Signals and Perception: The Fundamentals of Human Sensation. Basingstoke and New York: Palgrave Macmillan; 2002:3-16.
Axelsson A. Comparative anatomy of cochlear blood vessels. Am J Otolaryngol. 1988;9:278-290.
Blanks RH, Curthoys IS, Markham CH. Planar relationships of the semicircular canals in man. Acta Otolaryngol. 1975;80:185-196.
Bridger MW, Farkashidy J. The distribution of neuroglia and Schwann cells in the 8th nerve of man. J Laryngol Otol. 1980;94:1353-1362.
Chance SA, Casanova MF, Switala AE, Crow TJ. Minicolumnar structure in Heschl’s gyrus and planum temporale: asymmetries in relation to sex and callosal fiber number. Neuroscience. 2006;143:1041-1050.
Curthoys IS, Blanks RH, Markham CH. Semicircular canal radii of curvature (R) in cat, guinea pig and man. J Morphol. 1977;115:1-15.
Eybalin M. Neurotransmitters and neuromodulators of the mammalian cochlea. Physiol Rev. 1993;73:309-373.
Felix H, Hoffman V, Wright A, Gleeson MJ. Ultrastructural findings on human Scarpa’s ganglion. Acta Otolaryngol Suppl. 1987;436:85-92.
Fettiplace R. The transformation of sound stimuli into electrical signals. In: Roberts D, editor. Signals and Perception: The Fundamentals of Human Sensation. Basingstoke and New York: Palgrave Macmillan; 2002:17-28.
Fettiplace R, Hackney CM. The sensory and motor roles of auditory hair cells. Nat Rev Neurosci. 2006;7:19-29.
Furness DN. The vestibular system. In: Roberts D, editor. Signals and Perception: The Fundamentals of Human Sensation. Basingstoke and New York: Palgrave Macmillan; 2002:77-90.
Griffiths TD, Warren JD. What is an auditory object? Nat Rev Neurosci. 2004;5:887-892.
Guinan JJr. Physiology of olivocochlear efferents. In: Dallos P, Popper AN, Fay RR. The Cochlea. New York: Springer Verlag; 1996:435-502.
Comprehensive description of the efferent innervation of the cochlea and its function..
Keifer J, Böhnke F, Adunka O, Arnold W. Representation of acoustic signals in the human cochlea in presence of a cochlear implant electrode. Hearing Res. 2006;221:36-43.
Nadol JB. Comparative anatomy of the cochlea and auditory nerve in mammals. Hear Res. 1988;34:253-266.
Oertel D, Fay RR, Popper AN, editors. Integrative Functions in the Mammalian Auditory Pathway. Springer Handbook of Auditory Research, vol. 15. New York: Springer, 2002.
Ray B, Roy TS, Wadhwa S, Roy KK. Development of the human fetal cochlear nerve. Hearing Res. 2005;202:74-86.
Shepherd RK, Colreavy MP. Surface microstructure of the perilymphatic space. Implications for cochlear implants and cell- or drug-based therapies. Arch Otolaryngol Head Neck Surg. 2004;130:518-523.
Spoor F, Zonneveld F. Comparative review of the human bony labyrinth. Am J Phys Anthropol Suppl. 1998;27:211-251.
Wangemann P, Schacht J. Homeostatic mechanisms in the cochlea. In: Dallos P, Popper AN, Fay RR. The Cochlea. New York: Springer Verlag; 1996:130-135.
Warr WB. Organization of olivocochlear efferent systems in mammals. In: Webster DB, Popper AN, Fay RR. Mammalian Auditory Pathway: Neuroanatomy. New York: Springer Verlag; 1992:410-448.