Chapter 1
Indications for Pulmonary Function Testing
1. Categorize pulmonary function tests according to specific purposes.
2. List indications for spirometry, lung volumes, and diffusing capacity.
3. Identify at least one obstructive and one restrictive pulmonary disorder.
4. Relate pulmonary history to indications for performing pulmonary function tests.
1. Identify three indications for exercise testing.
2. Name at least two diseases in which air trapping may occur.
3. Describe the use of a technologist-adapted protocol for pulmonary function studies.
Pulmonary function tests
Many different tests are used to evaluate lung function. These tests can be divided into categories based on the aspect of lung function they measure (Box 1-1). Although the tests can be performed individually, they are often performed in combination. Figure 1-1 shows a sample pulmonary function test report that includes spirometry, lung volumes, diffusing capacity, and airway resistance measurements in a format that is commonly used. Determining which tests to do depends on the clinical question to be answered. This question may be explicit, such as, “Does the patient have asthma?” or less obvious, such as, “Does this patient, who needs thoracic surgery, have any pulmonary disease that might complicate the procedure?” In either case, indications for specific tests are useful (see Boxes 1-2 through 1-6).
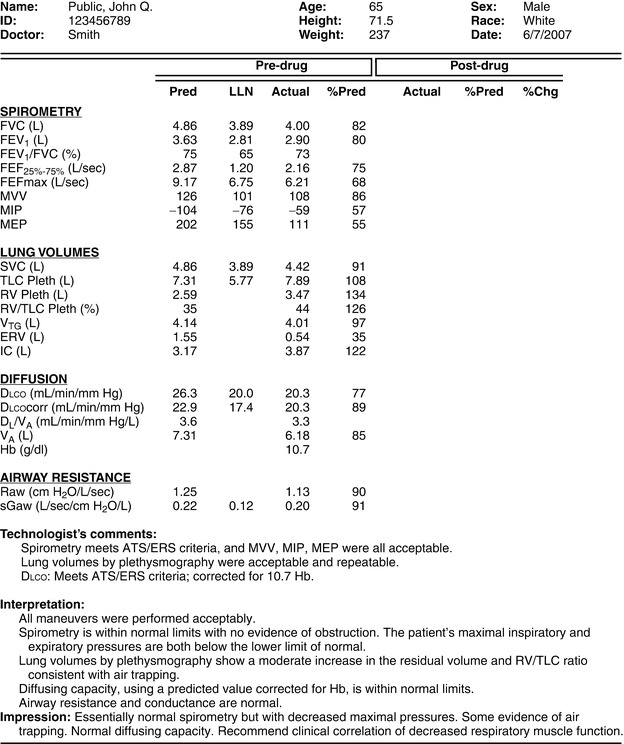
Lung function tests are grouped by category in the left column. The post-drug columns are blank because the patient was not retested after the bronchodilator.
LLN, Lower limit of normal.
Airway Function Tests
The most basic test of pulmonary function is the measurement of vital capacity (VC). This test simply measures the largest volume of air that can be moved into or out of the lungs. In the mid-1800s, a surgeon named Hutchinson developed a simple water-sealed spirometer that allowed measurement of what he named “vital capacity or vital breath” as he noted its relationship to survival. Hutchinson popularized the concept of using VC to assess lung function and named several other lung compartments that are still used today. He observed that VC was related to the standing height of the patient. He also developed tables to estimate the expected VC for a healthy patient. The VC was usually graphed on chart paper, which allowed subdivisions of the VC to be identified (see Chapter 2).
In addition to displaying FVC as a volume-time spirogram, it can also be represented by plotting airflow against volume. In the late 1950s, Hyatt and others began using the flow-volume display to assess airway function. The tracing was termed the maximal expiratory flow volume (MEFV) curve. By combining the forced expiration with an inspiratory maneuver, a closed loop can be displayed. This figure is called the flow-volume loop (see Chapter 2).
The FVC, FEV1, and other flows, along with flow-volume loops, are all used to measure response to bronchodilator medications (see Chapter 2). Tests are performed before and after inhalation of a bronchodilator, and the percentage of change is calculated. The same tests may be used to assess airway response after a challenge to the airways. These tests are referred to as bronchial challenge or bronchial provocation tests. The challenge may be in the form of a nonspecific inhaled agent (e.g., methacholine) or a physical agent (e.g., exercise). In either case, airflow is assessed before and after the challenge. The percent change (normally a decrease) after the challenge is calculated (see Chapter 9).
Airway resistance (Raw) measurements date back to the development of the body plethysmograph in the early 1950s. Comroe, DuBois, and others perfected a technique that provided estimates of alveolar pressure. The patient sits in an airtight box called a plethysmograph (see Chapter 11). The plethysmographic method allows the calculation of the pressure drop across the airways related to flow at the mouth (see Chapter 4). This technique originally required complicated monitoring and recording devices. Microprocessors have simplified the measurement of the required signals so that plethysmography is now widely used. The same equipment can also be used to rapidly and accurately measure thoracic gas volume (VTG).
Lung compliance is measured by passing a small balloon into the esophagus to measure pleural pressure. Intrapleural pressure can then be related to volume changes to estimate the distensibility of the lung (see Chapter 4). Other less invasive techniques are available but not widely used.
Lung Volume and Ventilation Tests
Closed-circuit (He dilution) and open-circuit (N2 washout) techniques are both widely used to measure FRC. Besides determining lung volumes, each technique provides some limited information about the distribution of ventilation within the lungs. The pattern of N2 washout can be displayed graphically. The time required for He to equilibrate during rebreathing provides a similar index of the evenness of ventilation. In the early 1950s and 1960s, Fowler developed a single-breath N2-washout technique. This method plotted N2 concentration in expired gas after a single breath of 100% oxygen. The single-breath N2 washout also provides limited information about gas distribution in the lungs. It also allows estimates of the lung volume at which airway closure occurs when the patient exhales completely (see Chapter 4).
Diffusing Capacity Tests
The basis for the modern single-breath diffusing capacity (Dlco) test was described by August and Marie Krogh in 1911. They showed that small but measurable differences existed between inspired and expired gas containing carbon monoxide (CO). This change could be related to the uptake of gas across the lung. Although they used the method to test a series of patients, they did not use the single-breath technique for clinical purposes. Around 1950, Forrester and others revisited the method. They developed it as a tool to measure the gas exchange capacity of the lung. About the same time, Filley and others were promoting other techniques, using CO to measure Dlco. Most of these techniques allowed patients to breathe normally, rather than hold their breath. These methods are called steady-state techniques. Each method has certain limitations. However, the single-breath technique is the most widely used and standardized in the United States and Europe (see Chapter 5).
Blood Gases and Gas Exchange Tests
Measurement of gases (O2 and CO2) in the blood began with volumetric methods used since the early 1900s. In 1957, Sanz introduced the glass electrode to measure pH of fluids potentiometrically. In 1958, Severinghaus added an outer jacket containing a bicarbonate buffer to the glass electrode. The electrode-buffer was separated from the blood being analyzed by a membrane that was permeable to CO2. This allowed the pressure of CO2 in the blood to be measured as a pH change in the electrode. In 1956, Leland Clark covered a platinum electrode with a polypropylene membrane. When a voltage was applied to the electrode, O2 was reduced at the platinum cathode in proportion to its partial pressure. These three electrodes (pH, Pco2, and partial pressure of oxygen [Po2]) were the basic measurement device in blood gas analyzers for many years. Miniature electrodes gradually replaced the traditional electrodes. Today, blood gas analyzers use a variety of electrochemical techniques (see Blood Gas Analyzers, Chapter 11) to measure not only pH, Pco2, and Po2, but also the various fractions of Hb, such as O2Hb and COHb. Similar methods to measure electrolytes (K++, Na++, Cl–) are also included in many blood gas analyzer systems. Transcutaneous electrodes, using techniques similar to the classical blood gas electrodes, are available for the measurement of O2 and CO2 tensions (tcpO2 and tcpCO2).
Capnography, or monitoring of exhaled carbon dioxide, was developed in conjunction with the infrared gas analyzer (see Chapter 11). This sensitive and rapidly responding analyzer allows exhaled CO2 to be monitored continuously. Most critical care units, operating rooms, and emergency departments use some combination of blood gas analysis, pulse oximetry, and capnography for patient monitoring. Blood gas analysis is an integral part of routine pulmonary function testing because it is the definitive test of the basic functions of the lung.
Indications for pulmonary function testing
Spirometry
Spirometry is the pulmonary function test performed most often because it is indicated in many situations (see Box 1-2). Spirometry is often performed as a screening procedure. It may be the first test to indicate the presence of pulmonary disease. Spirometry is recommended as the “gold standard” for diagnosis of obstructive lung disease by the National Lung Health Education Program (NLHEP), the National Heart Lung and Blood Institute (NHLBI), the World Health Organization (WHO), and numerous other organizations concerned with the diagnosis of lung diseases. However, spirometry alone may not be sufficient to completely define the extent of disease, response to therapy, preoperative risk, or level of impairment. Spirometry must be performed correctly because of the serious impact its results can have on the patient’s life. It is one of the few tests that yields a false-positive response if performed poorly because low values are the result. These low results may lead to further testing, inappropriate diagnosis, treatment, and increased costs for the patient and the health care delivery system.
Lung Volumes
Lung volume determination usually includes the VC and its subdivisions, along with the FRC. From these two basic measurements, TLC and other lung volumes can be calculated (see Chapter 4). Lung volumes are almost always measured in conjunction with spirometry, although the indications for them are distinct (see Box 1-3). The most common reason for measuring lung volumes is to identify restrictive lung disease. A reduced VC (or FVC) measured with spirometry may suggest restriction, particularly if airflow is normal. Measurement of FRC and determination of TLC are necessary to confirm restriction because a low FVC can be caused by either restriction or obstruction. If TLC is reduced below the 5th percentile of the predicted value, restriction is present. The severity of the restrictive process is determined by the extent of reduction of the TLC. TLC and its components can be determined by several methods. For patients with obstructive lung diseases (COPD, asthma), lung volumes measured by body plethysmography may be indicated (see Chapter 4) because multiple-breath or single-breath dilution techniques may underestimate TLC. In obstructive lung disease, lung volumes are necessary to determine whether air trapping or hyperinflation is present. The degree of hyperinflation, measured by indices such as the IC/TLC ratio, correlates with increased mortality in patients who have COPD.
Diffusing Capacity
Diffusing capacity is measured by having the patient inhale a low concentration of CO and a tracer gas to determine gas exchange within the lungs (Dlco). Several methods of evaluating the uptake of CO from the lungs are available, but the single-breath technique (Dlcosb) is most commonly used. This method is also called the breath-hold technique because CO transfer is measured during 10 seconds of breath holding. Dlco is usually measured in conjunction with spirometry and lung volumes. Although many pulmonary and cardiovascular diseases reduce Dlco (see Box 1-4), it may be abnormally increased in some cases (see Chapter 5). Dlco testing is commonly used to monitor diseases caused by dust (pneumoconioses). These are conditions in which lung tissue is infiltrated by substances such as silica or asbestos that disrupt the normal structure of the gas exchange units. Dlco testing is also used to evaluate pulmonary involvement in systemic diseases such as rheumatoid arthritis. Dlco measurements are often included in the evaluation of patients with obstructive lung disease, particularly in emphysema. Dlco tests may be indicated to monitor changes in lung function (i.e., gas exchange) induced by drugs used to treat cardiac arrhythmias, as well as changes caused by chemotherapy and radiation therapy for lung cancer.
Blood Gases
Blood gas analysis is the ideal measure of pulmonary function because it assesses the two primary functions of the lung (oxygenation and CO2 removal). Evaluation of many pulmonary disorders may include blood gas analysis. Specific indications for blood gas analysis are listed in Box 1-5. Blood gas analysis is most commonly used to determine the need for supplemental oxygen and to manage patients who require ventilatory support. Some pulmonary function measurements require blood gas analysis as an integral part of the test (i.e., shunt or dead space studies). Blood gas analysis is invasive; noninvasive measurements of oxygenation or gas exchange are often preferred since they are safer or less costly. Many noninvasive techniques (e.g., pulse oximetry, transcutaneous monitoring, and capnography) rely on a blood gas analysis to verify their validity (see Chapter 6).
Exercise Tests
Physical exercise stresses the heart, lungs, and the pulmonary and peripheral circulatory systems. Exercise testing allows simultaneous evaluation of the cellular, cardiovascular, and ventilatory systems. Cardiopulmonary exercise tests can be used to determine the level of fitness or extent of dysfunction. Appropriately designed tests can determine the role of cardiac or pulmonary involvement. COPD, interstitial lung disease, pulmonary vascular disease, and exercise-induced bronchospasm are respiratory disorders that often require exercise evaluation. Understanding the physiologic basis for the patient’s inability to exercise is an important aspect in prescribing effective therapy (i.e., cardiac or pulmonary rehabilitation). Exercise testing may also be required for the determination of disability. Box 1-6 lists specific indications for exercise tests.
Equipment used to measure oxygen consumption and CO2 production during exercise can also measure resting metabolic rates. This allows estimates of caloric needs in patients who are critically ill. Indications for performing studies of REE are detailed in Chapter 9.
Patterns of impaired pulmonary function
Obstructive Airway Diseases
Chronic Obstructive Pulmonary Disease
The term COPD is often used to describe long-standing airway obstruction caused by emphysema, chronic bronchitis, or asthma. These three conditions may be present alone or in combination (Figure 1-2). Bronchiectasis is sometimes considered a component of COPD. COPD is characterized by dyspnea at rest or with exertion, often accompanied by a productive cough. Delineation of the type of obstruction depends on the history, physical examination, and pulmonary function studies. Unfortunately, the term COPD is used to describe the clinical findings of dyspnea or cough without attention to the actual cause. This may lead to inappropriate therapy. Other similar terms include chronic obstructive lung disease (COLD) and chronic airway obstruction (CAO).
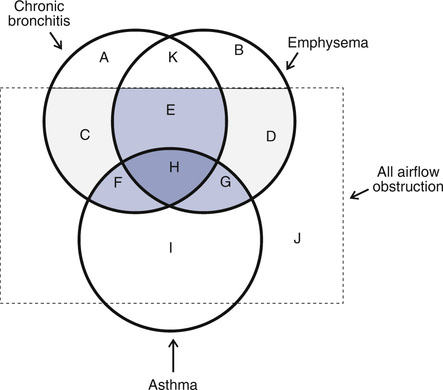
Emphysema, chronic bronchitis, and asthma overlap to varying degrees (shaded areas). Chronic obstruction in small airways and all airway obstructive diseases (large dashed square) also overlap. A, Patients with chronic bronchitis but no airflow obstruction. B, Patients with anatomic changes related to emphysema but no obstruction. C, Patients with chronic cough and airflow obstruction. D, Patients with emphysema and obstruction, as demonstrated by spirometry. E, Combined chronic bronchitis and emphysema, commonly occurring in the same patient, as a result of cigarette smoking. F, Combined chronic bronchitis and asthma. G, Combined emphysema and asthma. H, Combined asthma, chronic bronchitis, and emphysema. I, Patients with asthma manifested by reversible obstruction (spirometry or peak flow). J, Other forms of airway obstruction, including cystic fibrosis, bronchiolitis obliterans, or upper airway abnormalities (e.g., vocal cord dysfunction), are not considered part of COPD. K, Patients with cough and morphologic evidence of emphysema but no obstruction. (Modified from American Thoracic Society. Standards for the diagnosis and care of patients with chronic obstructive pulmonary disease. Am J Respir Crit Care Med. 1995; 152:S77-S120.)
Emphysema
The chest x-ray film of a patient with emphysema shows flattened diaphragms and increased air spaces. The lung fields appear hyperlucent (dark) with little vascularity. The heart appears to be hanging from the great vessels (Figure 1-3). Computerized tomography (CT) scans, especially spiral CT scans, show a three-dimensional picture of enlarged air spaces and loss of supporting tissue. CT scans also delineate whether the emphysematous changes are localized or spread throughout the lungs.
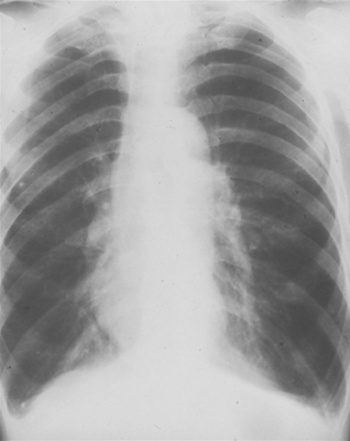
The physical appearance of the chest confirms what is shown radiographically. The chest wall is immobile with the shoulders elevated. The diameter of the chest is increased in the anterior-posterior aspect (so-called barrel chest). There is little diaphragmatic excursion during inspiration. Intercostal retractions may be prominent. Accessory muscles (neck and shoulders) are used to lift the chest wall. Breath sounds are distant or absent. Patients may need to support the arms and shoulders to catch their breath. Breathing is often done through pursed lips in an attempt to alleviate the sensation of dyspnea (Figure 1-4).
Management of Chronic Obstructive Pulmonary Disease
The Global Initiative for Chronic Obstructive Lung Disease (GOLD), a World Health Organization(WHO) program, works with health care professionals to raise awareness and to improve the prevention and treatment of COPD. COPD is the third leading cause of morbidity and mortality throughout the world. COPD often includes components of emphysema and chronic bronchitis (see Figure 1-2). This association most likely is due to the common risk factor of cigarette smoking. Hyperreactive airways disease (asthma) may also be present. Reversibility of obstruction, however, is usually less than in uncomplicated asthma. Bronchiectasis and bronchiolitis are also commonly found in patients with COPD.
Breathing retraining, bronchial hygiene measures, and physical reconditioning are important therapeutic modalities in addition to pharmacologic management. Breathing retraining is especially important for the patient with advanced COPD. Grossly altered pulmonary mechanics favor hyperinflation and the use of accessory muscles. Training in the use of the diaphragm for slow, relaxed breathing can significantly improve gas exchange. Pulmonary rehabilitation, particularly physical reconditioning, permits many patients with otherwise debilitating disease to maintain their quality of life (Figure 1-5).
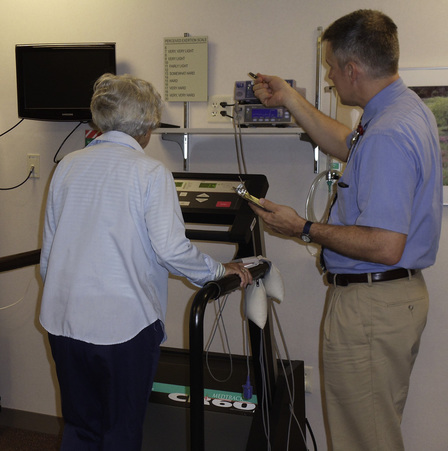
Single-lung transplantation has been used for patients with end-stage COPD who are younger than 60 years old. Although lung transplantation causes immediate improvement in pulmonary function, there are factors that need to be considered. The cost of hospitalization and follow-up care is extensive, and the lack of donor organs means that many patients with COPD die while awaiting transplantation. The prognosis for those receiving lung transplants is generally good. In some transplant recipients, a severe form of airway obstruction (bronchiolitis obliterans) has been found to occur in the transplanted lung. The reason for this obstructive process is unclear, but the progression is rapid. Spirometry is used to monitor transplant recipients to detect early changes associated with bronchiolitis obliterans. (See Figure 1-6).
Hyperreactive Airways Disease: Asthma
Asthma is characterized by reversible airway obstruction. Obstruction is caused by inflammation of the mucosal lining of the airways, bronchospasm, and increased airway secretions. Bronchospasm is usually reversed by the inhalation of bronchodilators but may be persistent and severe in some patients. Inflammation is the essential element in the asthmatic response. Increased airway responsiveness is related to the inhalation of antigens, viral infections, air pollution, occupational exposure, cold air, and exercise. Spirometry is the most useful tool for detecting reversible airway obstruction. Improvement in the FEV1 or FVC (see Chapter 2) is the hallmark of reversibility. Airway resistance (Raw) and specific airway conductance (SGaw) are also useful in the evaluation of reversible obstruction. Peak expiratory flow (PEF), measured using portable peak flow meters, can provide immediate information for a clinician or patient to modify therapy. Analysis of eNO can detect inflammatory changes in the airways even in the absence of spirometric or peak flow abnormalities.
Asthma can occur at any age but often begins during childhood. Even infants can have hyperreactive airways (see Chapter 8). Some asthmatic children outgrow the disease, but, in others, the disease continues into adulthood. In some individuals, asthma begins in adulthood, usually after age 40. There appears to be a hereditary component to asthma; many cases occur in patients who have a family history of asthma or allergic disorders.
Agents or events that cause an asthmatic episode are called triggers (Box 1-7). Antigens such as animal dander, pollens, and dusts are the most common triggers. Other common triggers include exposure to air pollutants, exercise in cold or dry air, occupational exposure to dusts or fumes, and viral upper respiratory infections. Aspirin or other drugs can also trigger asthma, as can food additives (e.g., metabisulfites), or emotional upset (e.g., crying, laughing). All of these triggers act on the hyperresponsive airway to produce the symptoms of asthma.
Management of Asthma
A significant tool in the management of asthma is the portable peak flow meter (see Chapters 2 and 11). This device allows simple monitoring of airway function by the patient at home, as well as by caregivers in a variety of settings. Measuring peak flow provides objective data to guide both the patient and physician in modifying bronchodilator therapy or seeking early treatment. Computerized peak flow meters that include symptom history (i.e., an electronic diary) allow asthma management to be tailored to the individual patient’s asthmatic needs.
Upper or Large Airway Obstruction
Many obstructive diseases involve the medium or small airways. Sometimes airway obstruction occurs in the upper airways (nose, mouth, or pharynx) or in the large thoracic airways (trachea, main stem bronchi). Obstruction can also occur where the upper and lower airways meet at the vocal cords. When obstruction occurs below the vocal cords, the degree of obstruction may vary with changes in thoracic pressure. This occurs because the airways themselves change size as thoracic pressure rises or falls. Obstructive processes above the vocal cords are not influenced by thoracic pressures but may still vary with airflow, depending on the type of lesion involved. Regardless of the location of the problem, large airway obstruction results in increased work of breathing. Extrathoracic or intrathoracic airway obstruction is frequently diagnosed using the flow-volume loop or measurements of airway resistance (see Chapter 4).
Vocal cord dysfunction or damage can result in significant airway obstruction. The vocal cords are normally held open or abducted during inspiration. When damaged, the vocal cords move toward the midline, narrowing the airway opening. This type of obstruction limits flow primarily during inspiration. In some cases, expiratory flow may be reduced as well, but inspiratory flow is typically lower. Common causes of vocal cord dysfunction (VCD) include laryngeal muscle weakness or mechanical damage, as sometimes occurs during intubation of the trachea. Severe infections involving the larynx can leave scar tissue on the vocal cords or supporting structures. Vocal cord dysfunction often mimics asthma. It may become noticeably worse when ventilation is increased, as happens during exercise. Neuromuscular disorders can cause paralysis of the vocal cords, also resulting in variable extrathoracic airway obstruction (see Chapter 2).
Tumors are a common cause of large airway obstruction. Lesions that invade the trachea or main stem bronchi can significantly diminish airflow. The decrease in flow is directly related to the decrease in cross-sectional area of the airway. If the airway lumen (i.e., the part not obstructed) varies in cross-sectional area with inspiration and expiration, the obstruction is described as variable. During inspiration, thoracic pressure decreases and large airways increase their cross-sectional area. During expiration, the opposite occurs. If the airway is partially obstructed by a tumor, airflow will be decreased during inspiration and expiration but more so during expiration. If the tumor reduces the cross-sectional area of the airway but does not cause it to change with the phase of breathing, the obstruction is fixed. In this instance, both inspiratory and expiratory flows are reduced approximately equally (see Chapter 2). Tumors involving the upper airway may cause variable or fixed obstruction. If an extrathoracic tumor causes the airway cross section to vary with breathing, inspiratory flow is usually reduced. An easy way to remember the cause and effect is to use the mnemonic, “What’s in is out, what’s out is in.” In other words, if the obstruction is within the thoracic cage, it will affect the expiratory flow volume curve. Whereas, if the obstruction is outside of the thoracic cage (i.e., VCD), it will affect the inspiratory portion of the flow volume loop.
Management of Upper or Large Airway Obstruction
Treatment of extrathoracic or intrathoracic large airway obstruction is aimed at reversing the process produced by the offending lesions. For vocal cord dysfunction, stopping inappropriate therapy (e.g., steroids) is the first step. Speech therapy and breathing retraining have been demonstrated to reduce inspiratory obstruction. In severe cases, a mixture of helium and oxygen (80% He–20% O2) may be needed to alleviate dyspnea and interrupt the episode. Treatment of neuromuscular disease, such as myasthenia gravis, often reverses the associated airway obstruction. Tumors usually require resection. Some neoplasms can be managed by radiation or chemotherapy only. In either case, spirometry with flow-volume curves (see Chapter 2) is used to assess airway obstruction. Surgical repair of trauma to the upper or large airways directly relieves airway obstruction and reduces the work of breathing.
Restrictive Lung Disease
• interstitial lung diseases, including idiopathic fibrosis, pneumoconioses, and sarcoidosis
• disease of the chest wall and pleura
• congestive heart failure (CHF)
• scarring (fibrosis) caused by radiation or chemotherapy
• transient problems such as pleural effusions, abdominal ascites, or pregnancy
Pulmonary Fibrosis
Pulmonary fibrosis often follows the use of medications such as bleomycin, cyclophosphamide, methotrexate, or amiodarone. It is also associated with a number of autoimmune diseases. Rheumatoid arthritis, systemic lupus erythematosus (SLE), and scleroderma all produce alveolar wall inflammation and fibrotic changes. As each disease progresses, lung volumes are reduced. These reductions in VC and TLC occur as fibrosis causes the lungs to become stiff. Measurement of pulmonary compliance (see Chapter 2) is sometimes helpful in quantifying the effects of the fibrosis. Dlco (see Chapter 3) is often reduced because of loss of lung volume and ventilation-perfusion mismatching. The same processes also cause hypoxemia at rest that worsens with exertion.
Pneumoconioses
Pneumoconiosis is lung impairment caused by inhalation of dusts. Specific types of dust exposures have been shown to result in pneumoconioses (Table 1-1). Dust particles in the size range between 0.5 and 5.0 microns are considered most dangerous because they are deposited throughout the lung. A carefully taken history of the patient’s exposure, including work history, is essential. (See Pulmonary History in the Preliminaries to Patient Testing section). Most pneumoconioses are characterized by pulmonary fibrosis and chest x-ray abnormalities. Pulmonary function studies typically reveal a restrictive pattern with reduction in Dlco.
Table 1-1
Dust | Pneumoconiosis | Occupation |
Iron | Siderosis | Welder, miner |
Tin | Stannosis | Metal worker |
Barium | Baritosis | Miner, metallurgist, ceramics worker |
Silica | Silicosis (aka Grinder’s disease or Potter’s rot) | Sandblaster, granite worker, brick maker, coal miner |
Asbestos | Asbestosis | Brake/clutch manufacturer, shipbuilder, steam fitter, insulator |
Talc | Talcosis | Ceramics worker, cosmetics maker |
Beryllium | Berylliosis | Alloy maker, electronic tube maker, metal worker |
Coal | Coal worker’s pneumoconiosis | Coal miner |
Neuromuscular Disorders
Myasthenia gravis is a chronic autoimmune disease affecting neuromuscular transmission. In myasthenia gravis, antibodies produced by the body’s immune system block or destroy the receptors for acetylcholine at the neuromuscular junction which prevents the muscle contraction from occurring. It particularly affects muscles innervated by the bulbar nuclei (i.e., face, lips, throat, and neck). The patient with myasthenia gravis has pronounced fatigability of the muscles. Speech and swallowing difficulties can occur with prolonged exercise of the associated muscles. Administration of edrophonium chloride (Tensilon) is sometimes used to confirm the diagnosis of myasthenia gravis; the drug blocks acetylcholinesterase and temporarily increases muscle strength in patients who have myasthenia gravis. When the ventilatory muscles become involved, a myasthenic crisis occurs. Progression of a myasthenic crisis can be assessed using VC and respiratory pressures. Analysis of the flow-volume curve (see Chapter 2) may be helpful in detecting upper airway obstruction brought on by muscular weakness.
Lung Transplantation
Lung transplantation has evolved as an effective treatment for end-stage lung disease. Lung transplantation has been used for patients with CF, primary pulmonary hypertension, and COPD (Table 1-2). Double-lung transplants are usually performed in patients who have CF, generalized bronchiectasis, or in some types of COPD. Heart-lung transplants have been used for Eisenmenger’s syndrome, pulmonary hypertension with cor pulmonale, and end-stage lung disease coexisting with severe heart disease. Single-lung transplantation has been used effectively in patients with COPD who are younger than approximately 60 years old. Single-lung transplantation offers the benefit that two recipients can share a single donor’s organs. Survival rates for lung transplant recipients have steadily improved. Longer survival is mainly due to more potent anti-rejection drugs (e.g., cyclosporine) and better adjunctive therapy. Pulmonary function tests are used to both assess potential transplant candidates and follow them postoperatively.
Table 1-2
Indications for Lung Transplantation*
Transplant Type | Disease State |
Heart-lung | Eisenmenger’s syndrome, severe cardiac defect Pulmonary hypertension, cor pulmonale End-stage lung disease, coexisting severe cardiac disease |
Double-lung | Cystic fibrosis Generalized bronchiectasis COPD with severe chronic bronchitis or extensive bullae |
Single-lung | Restrictive fibrotic lung disease Eisenmenger’s syndrome (less severe cardiac anomalies) COPD Primary pulmonary hypertension |
*Modified from American Thoracic Society. Lung transplantation. Am Rev Respir Dis. 1993; 147:772-776.
Preliminaries to patient testing
Before Patient Testing
In order to provide accurate patient data, the laboratory needs to have a defined quality assurance program in place. The Clinical and Laboratory Standards Institute (CLSI), an organization that provides guidance for medical laboratories, adapted the concepts found in International Standards Organization (ISO) 9001 recommendations and applied them to the laboratory setting in a guideline titled, “A Quality Management System for Healthcare.” This guideline describes the various hierarchical stages of quality (Table 1-3) where a quality management system is a systematic “process-oriented” approach to quality. The quality system model describes, documents, implements, measures, and monitors the implementation and effectiveness of the work operations of the laboratory. The model is characterized by quality elements that are essential components of an organization or laboratory’s quality system and are called “the quality system essentials,” or “QSEs.”
Table 1-3
The QMS is a major level in the health care quality hierarchy and forms the basis for this document. Also see Figure 1-7.1 | |
Stage | Activities Performed |
Total Quality Management | Management approach centered on sustained high quality, by focusing on long-term success through customer satisfaction |
Quality Cost Management | Measurement system for the economic aspects of the “cost of quality” |
Quality Management System | Systematic process-oriented approach to meeting quality objectives |
Quality Assurance | Planned and systematic activities to provide confidence that an organization fulfills requirements for quality |
Quality Control | Operational process control techniques to fulfill quality requirements for regulatory compliance and accreditation2 |
1Cianfrani CA, Tsiakals JJ, West JE. The ASQ ISO 9000:2000 Handbook, Milwaukee WI: American Society for Quality; 2002.
2ISO: Quality management systems: Fundamentals and vocabulary. ISO 9000. Geneva, Switzerland: International Organization for Standardization; 2005.)
(From Clinical and Laboratory Standards Institute (CLSI). Quality management system: A model for laboratory services, approved guideline. 4th ed. CLSI document GP26-A4, Wayne PA: Clinical and Laboratory Standards Institute; 2011.
In CLSI’s quality system model, these 12 QSEs are identified across the laboratory’s “Path of Workflow.” In the pulmonary function laboratory, the path of workflow (POW) would include pre-test, test, and post-test activities. Chapter 12 will further detail the POW activities and how integrating these concepts into a laboratory’s quality plan ensures quality testing results (Figure 1-7).
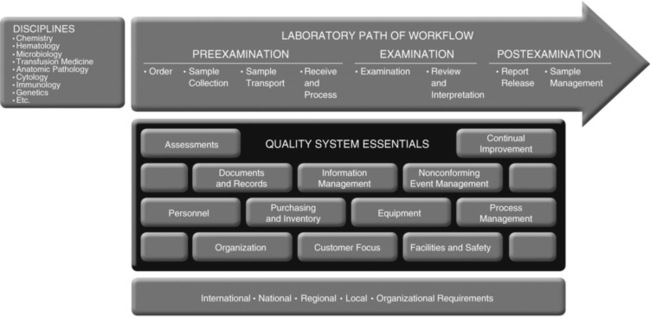
This example represents how the 12 QSEs support a clinical laboratory’s disciplines. (Clinical and Laboratory Standards Institute [CLSI]. Quality management system: A model for laboratory services, approved guideline. 4th ed. CLSI document GP26-A4, Wayne PA: Clinical and Laboratory Standards Institute; 2011.)
Withholding Medications
Patients referred for evaluation of airflow limitation are often already taking bronchodilators or related drugs. If response to bronchodilators is to be assessed, bronchodilators should be withheld before testing. The exact length of time to withhold a bronchodilator is dictated by the onset of action and how long it takes for the drug to be metabolized and/or excreted. Guidelines for withholding specific bronchodilators before simple spirometry are presented in detail in Chapter 2. Recommendations for withholding medications before a bronchial challenges test (methacholine, exercise, hyperventilation, etc.) are found in Chapter 9. Some patients may have difficulty withholding bronchodilators. In the case of simple spirometry, the test may be performed if necessary, with a technologist comment describing the use of bronchodilators before testing. The patient should be instructed to take the bronchodilator when breathing problems require it. Patients scheduled for a bronchial challenge who inadvertently take their bronchodilators may need to be rescheduled because bronchodilators can significantly alter airway response and lead to false-negative results.
Smoking Cessation
Patients referred for pulmonary function tests should be asked to refrain from smoking for 24 hours before the test. Smoking cessation is especially important if Dlco tests or arterial blood gas tests are ordered. Smoking has been shown to directly reduce Dlco. Smoking also raises the level of CO in the blood, which also interferes with the measurement of Dlco (CO back pressure in blood). Increased CO in the blood (COHb) also makes it difficult to interpret O2 saturation measured by pulse oximetry (see Chapter 6). Smoking has been shown to reduce the levels of eNO, which may artifactually reduce the level of NO in patients who have airway inflammation.
Other Patient Preparation Issues
Patients referred for pulmonary function tests should refrain from eating a large meal immediately before their appointment. Two hours is usually sufficient to avoid vomiting or gastric distress during routine testing. The same is true if the patient will be exercising as part of the evaluation. Patients scheduled for a bronchial challenge test (see Chapter 9) should not drink beverages that contain caffeine or cola (theobromines) or eat chocolate. Outpatients scheduled for metabolic studies may need to fast for 8 hours before testing to assure a stable baseline. Patients should refrain from alcohol consumption for at least 4 hours before testing (see Box 1-8).
Anthropometric Measurements
Standing height, in either inches or centimeters (to the nearest ¼ inch or 0.5 cm), should be recorded with the patient barefoot or in stocking feet. A wall-mounted ruler (stadiometer) allows the patient to stand with the back against the wall and the head close to the ruler. If the patient is unable to stand upright, the arm-span method should be used. Patients who have a history of kyphosis, scoliosis, or related problems should also have the height estimated using their arm span. Arm span may be measured using a ruler placed on a wall or a tailor’s tape measure (Figures 1-8, A-C). The patient should extend the arms horizontally on both sides, and the distance between the tips of the middle fingers measured. Alternately, the distance from the tip of the middle finger to the center of the vertebrum at the level of the scapula is measured on each side and then summed. Height may then be estimated as arm span/1.06 or may be estimated using regression equations that account for race, sex, and age, in addition to arm span.
The patient’s weight in pounds or kilograms should be measured with an accurate scale. Although body weight is typically not used in the calculation of predicted lung volumes, subjects that are morbidly obese may demonstrate a restrictive pattern. Measurement of weight and calculation of BMI may be helpful for the interpretation of reduced lung volumes (e.g., FRC, ERV). Body weight is used to calculate other reference values (see Chapter 13). When weight is used to predict an expected value, the patient’s ideal body weight should be used, unless noted otherwise. Using actual weight in patients who are obese may overestimate expected values if the reference set is based on subjects with normal weights. Weight is also used to express oxygen consumption (i.e., milliliter/kilogram) for exercise and metabolic measurements. The patient’s weight may also be required when lung volumes are determined with the body plethysmograph. Weight is used to estimate body volume in the plethysmograph (see Chapter 4).
Physical Assessment
Physical assessment of patients referred for pulmonary function studies may be needed to determine whether the individual can perform the test. Documentation concerning physical assessment of the patient can also assist with interpretation of test results. Physical assessment should focus on breathing pattern, breath sounds (if necessary), and respiratory symptoms (Box 1-9). These can be observed simply and noted as necessary. Assessment of the patient’s oxygen status using pulse oximetry (Chapter 6) may be required, especially if the subject presents to the laboratory using oxygen therapy. Some test modalities require the patient to be off oxygen, and if the patient is intolerant, that portion of the test will not be performed. Documenting technologist/therapist comments regarding the patient’s signs, symptoms, and oxygenation status at the time of the test is a useful adjunct, especially if test performance is less than optimal.
Pulmonary History
Accurate interpretation of pulmonary function studies—from simple screening spirometry to complete cardiopulmonary evaluation—requires clinical information related to possible pulmonary disease. An ordered array of questions that can be easily answered by the patient provides the most useful history. The interpreter of pulmonary function studies may have little clinical information other than that obtained at the time of testing. A pulmonary history should be taken routinely before pulmonary function testing (Box 1-10).
Test performance and sequence
Pulmonary function laboratories should have written policies and procedures defining how each test is performed (see Chapter 12). An excellent resource is the American Thoracic Society’s Pulmonary Function Laboratory Management and Procedure Manual, which is available on their website. Indications for performing a specific test should be related to the clinical question to be answered or to the patient’s diagnosis. Testing protocols that can be modified for individual patients are usually the most cost-effective means of obtaining the required data. When the required tests have been determined, the exact sequence of tests can be selected. The sequence in which tests are performed may vary according to patient need and the test method used. For example, a patient with severe obstructive lung disease may not be able to perform an acceptable Dlco maneuver until a bronchodilator has been administered.
Technologist-Driven Protocols
As described previously, the basis for deciding which pulmonary function tests are needed is related to the clinical question being asked. The clinical question is often inappropriately stated as a diagnosis. In fact, many patients are referred for pulmonary function studies to establish a diagnosis. For example, a patient may be referred with a diagnosis listed as “asthma.” The clinical question is, “Does the patient have asthma?” Pulmonary function studies may be able to help answer this question, but the exact tests to be performed may not be defined. In this example, spirometry is indicated. Using an adaptive protocol, spirometry can be performed and, based on the results, appropriate additional tests selected (Figure 1-9). Bronchial challenge tests may be performed if spirometry results are normal. Alternatively, additional tests such as lung volumes or Dlco may be necessary.
Patient Instruction
Even after adequate instruction and demonstration, some patients may be unable to perform certain tests. This may be caused by lack of coordination related to illness, pain as a result of their condition, or inability to follow instructions. For example, a patient may experience uncontrollable coughing when asked to inspire deeply for an FVC maneuver. If the coughing prevents obtaining valid spirometry results, the fact should be noted in the technologist’s comments (see Chapter 12). Suboptimal effort by the patient can usually be detected as poorly repeatable results on effort-dependent tests (e.g., the FVC). Care should be taken that adequate instructions are given and a sufficient number of efforts recorded before deciding that the patient did not give a maximal effort. If the patient cannot continue or refuses to continue a test, the exact reason should be documented in the technologist’s comments.
Summary
• Tests are categorized as airway function tests (spirometry), lung volume tests, Dlco tests, blood gases and gas exchange tests, cardiopulmonary exercise tests, and other specialized tests. Within each of these groups is a wide variety of tests and techniques.
• Indications are extremely important because they help the practitioner select appropriate tests. The clinical question asked of the test must be related to a valid indication for the test.
• Underlying pathology involved in common pulmonary diseases and the role of pulmonary function testing is discussed as it relates to diagnosis and assessment of various diseases.
• Patient preparation for pulmonary function studies is covered in general terms. More detailed information for specific tests is presented in subsequent chapters. Many of the physical measurements and assessments, as well as the pulmonary history, are similar regardless of the tests being performed. Technologist-driven protocols are described. Algorithms for selecting only appropriate tests are becoming increasingly popular. Such tools improve the sensitivity of the tests to answer the clinical question, as well as make tests more cost-effective.