10 IMMUNE-LYMPHATIC SYSTEM
Organization of the immune-lymphatic system
The lymphatic system includes primary and secondary lymphoid organs.
The primary lymphoid organs produce the cell components of the immune system. They are (1) the bone marrow and (2) the thymus. The main cell type is the lymphocyte originated from a lymphoid stem cell in bone marrow (Figure 10-1).
The main function of the lymphoid organs, as components of the immune system, is to protect the body against invading pathogens or antigens (bacteria, viruses, and parasites). The basis for this defense mechanism, or immune response, is the ability to distinguish self from nonself.
The two key cell components of the immune system are lymphocytes and accessory cells (Figure 10-2). Lymphocytes include two major cell groups: (1) B cells, responding to cell-free and cell-bound antigens; and (2) T cells, subdivided into two categories: helper T cells and cytolytic or cytotoxic T cells. T cells respond to cell-bound antigens presented by specific molecules.
INNATE (NATURAL) AND ADAPTIVE (ACQUIRED) IMMUNITY
Immunity in general is the reaction of cells and tissues to foreign (nonself) substances or pathogens including bacterial, viral, and parasite antigens. Two types of immunity are distinguished: (1) innate or natural immunity and (2) adaptive or acquired immunity (Figure 10-3).
Innate or natural immunity of the newborn is the simplest mechanism of protection. It does not require previous exposure to a pathogen and elicits rapid responses by macrophages and dendritic cells. Toll-like receptors (see Box 10-A) initiate innate immunity against components of invading pathogens (such as nucleic acids, proteins, lipids and polysaccharides). Stimulation of macrophages and dendritic cells by their ligand-bound Toll-like receptors leads to the production and secretion of proinflammatory cytokines, thereby initiating an inflammatory response.
Box 10-A Toll-like receptors
Adaptive or acquired immunity develops when an individual is exposed to a pathogen with the aims of eliminating the pathogen as well as the generation of immunologic memory. To achieve adaptive or acquired immunity, it is necessary to select lymphocytes (clonal selection) from a vast repertoire of cells bearing antigen-specific receptors generated by a mechanism known as gene rearrangement. Essentially, adaptive immunity is the perfection of innate or natural immunity that recognizes vital components of the microorganism utilizing a limited number of pattern-recognition receptors expressed on all cells of a given type (nonclonal) and independent of immunologic memory.
Adaptive immunity involves two types of responses to an antigen (pathogen): The first response is mediated by antibodies produced by plasma cells, the final differentiation product of B cells as we have seen in Chapter 4, Connective Tissue. This response is known as humoral immunity and operates against antigens located outside a cell or bound to its surface. When antibodies bind to an antigen or toxins produced by a pathogen, they can facilitate the phagocytic action of macrophages or recruit leukocytes and mast cells to take advantage of their cytokines and mediators, respectively, and strengthen a response. Humoral immunity results in persistent antibody production and production of memory cells.
Properties of adaptive or acquired immunity
B CELLS
The bone marrow is the site of origin of B and T cells from a lymphoid stem cell. In Chapter 6, Blood and Hematopoiesis, we discussed developmental aspects of the myeloid and erythroid lineages from a hematopoietic stem cell. The same hematopoietic stem cell gives rise to a lymphoid stem cell that generates precursors for B cells and T cells (see Figure 10-1). B cells mature in the bone marrow, whereas the thymus is the site of maturation of T cells.
Stem B cells in the bone marrow proliferate and mature in a microenviron-mental niche provided by bone marrow stromal cells producing interleukin-7 (IL-7) (Figure 10-4). During maturation, B cells express on their surface immunoglobulins M (IgM) or D (IgD) interacting with two additional proteins linked to each other, immunoglobulins α (Igα) and β (Igβ). The cell surface IgM or IgD, together with the conjoined Igα and Igβ, form the B cell antigen receptor complex. The intracellular domains of Igα and Igβ contain a tyrosine-rich domain called immunoreceptor tyrosine-based activation motif (ITAM).
Self-antigens present in the bone marrow test the antigen-binding specificity of IgM or IgD on B cell surfaces. This is a required testing step before B cells can continue their maturation, enter peripheral lymphoid tissues, and interact with foreign (non-self) antigens. Self-antigens binding strongly to two or more IgM or IgD receptor molecules on B cells induce apoptosis. Self-antigens with a weaker binding affinity for the B cell antigen receptor complex enable the survival and maturation of these B cells when ITAMs of IgM- or IgD-associated Igα and Igβ transduce signaling events, resulting in further differentiation of B cells and the entrance of mature B cells into the circulation.
T CELLS
Major histocompatibility complex and human leukocyte antigens
The presentation of antigens to T cells is carried out by specialized proteins encoded by genes in the major histocompatibility locus and present on the surface of antigen-presenting cells. Antigen-presenting cells survey the body, find and internalize antigens by phagocytosis, break them down into antigenic peptide fragments, and bind them to major histocompatibility complex (MHC) molecules (Figure 10-5) so that the antigen peptide fragment–MHC complex can be exposed later on the surface of the cells. The MHC gene locus expresses gene products responsible for the rejection of grafted tissue between two genetically incompatible hosts.
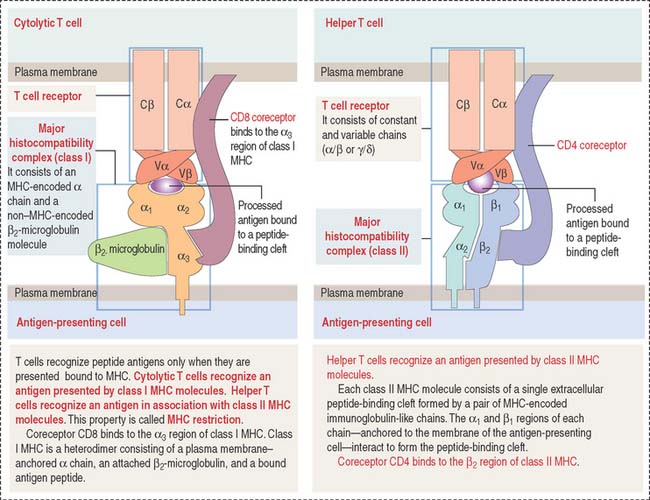
Figure 10-5 Structure of the T cell receptor and class I and II major histocompatibility complex (MHC)
Class II MHC consists of two polypeptide chains, an α chain and a β chain. Both chains are encoded by the MHC gene locus. The α1 and β1 domains form an antigen-binding cleft. CD4, a coreceptor on the surface of helper T cells, binds to the β domain of class II MHC. CD4 and CD8 are cell surface identifiers, members of the cluster of differentiation or designation (abbreviated as CD). See Box 10-B.
Box 10-B CD antigens
T cell receptor complex
In addition to MHC molecules, subsets of T cells have cell surface receptors that enable each of them to recognize a different antigen peptide–MHC combination. Antigen recognition involves an immunologic synapse mechanism consisting in the formation of stable antigen-presenting cell–T cell adhesiveness followed by an activating signaling cascade by T cells (see Box 10-C).
Box 10-C The Immunologic synapse
The TCR consists of two disulfide-linked transmembrane polypeptide chains: the α chain and the β chain (see Figure 10-5). A limited number of T cells have a TCR composed of γ and δ chains. Each α and β chain consists of a variable (Vα and Vβ) domain and a constant (Cα and Cβ) domain. When compared with the immunoglobulin molecule, the Vα and Vβ domains are structurally and functionally similar to the antigen-binding fragment (Fab) of immunoglobulins.
The TCR molecule is associated with two proteins, CD3 and ζ (not shown in Figure 10-5), forming the TCR complex. CD3 and ζ have a signaling role and are present in all T cells. CD3 contains the ITAM cytoplasmic domain previously mentioned as part of the B cell antigen receptor complex and involved in signaling functions.
CD4 and CD8 coreceptors
CD4 and CD8 are T cell surface proteins interacting selectively with class II MHC and class I MHC molecules, respectively. When the TCR recognizes an antigen bound to the cleft of MHC, CD4 or CD8 coreceptors cooperate in the activation of T cell function (see Figure 10-5).
CD4 and CD8 are members of the immunoglobulin (Ig) superfamily. In Chapter 1, Epithelium, we discussed the function and structure of cell adhesion molecules belonging to the Ig superfamily.
Members of the Ig superfamily have a variable number of extracellular Ig-like domains. The two terminal Ig-like domains of CD4 bind to the β2 domain of the class II MHC (see Figure 10-5). The single Ig-like domain of CD8 binds to the α3, domain of the class I MHC.
Thus, CD4+ helper T cells recognize antigens associated with class II MHC, and CD8+ cytolytic T cells (cytolytic thymus-derived lymphocytes [CTL]) respond to antigens presented by class I MHC (Figure 10-6).
MHC molecules and adaptive immune responses
During their maturation in the cortex of the thymus, T cells are selected to be self-MHC–restricted and self-tolerant. This selective process, known as positive selection, occurs only when self-MCH–restricted T cells are selected (see Figure 10-7). Negative selection takes place when T cells do not bind to any MHC or bind to the body’s tissue-specific antigens (self-molecules). We discuss later how a portfolio of self-antigens expressed in the thymus permits the elimination of autoreactive T cells by apoptosis. Only those T cells that can recognize foreign peptides and self-MHC survive, leave the thymus, and migrate into the secondary lymphoid organs.
The cortex of the thymus contains branching and interconnected thymic cortical epithelial cells involved in the positive selection of T cells. The medulla of the thymus houses thymic medullary epithelial cells involved in the negative selection of potentially autoreactive T cells. Contact between MHC molecules on the thymic epithelial cell surfaces and TCRs of developing T cells is an important feature in positive selection. This is another example of the importance of the immunological synapse (see Box 10-C).
T cells developing in the thymus express specific cell surface molecules
TCR consists of two pairs of subunits: αβ chains or γδ chains (see Figure 10-3). Each chain can vary in sequence from one T cell to another. This variation is determined by the random combination of gene segments and has a bearing on which foreign antigen T cells can recognize.
T cell-mediated immunity
Cytolytic (or killer) T cells display both the TCR and CD8 coreceptor. Cytolytic T cells recognize class I MHC on antigen-presenting cells. We will return to the clinical significance of helper and cytolytic T cells when we discuss their involvement in the pathology of human immunodeficiency virus-type 1 (HIV-1) infection, allergy, and cancer immunotherapy.
How do helper T cells help?
Helper T cells are activated when they recognize the antigen peptide–class II MHC complex (Figure 10-8).
Plasma cells synthesize only one class of immunoglobulin (several thousand immunoglobulin molecules per second; lifetime of a plasma cell is from 10 to 20 days). Five classes of immunoglobulins are recognized in humans: IgG, IgA, IgM, IgE, and IgD (see Box 10-D). Abnormal plasma cells may accumulate in bones and bone marrow, causing bone destruction and affecting the production of normal blood cells. This pathologic condition is called multiple myeloma (see Box 10-E).
Box 10-D Immunoglobulins
Box 10-E Multiple myeloma
How do cytolytic T cells kill?
The subset of cytolytic T cells initiates a target cell destruction process (Figure 10-9) by (1) attaching firmly to the antigen-presenting cell with the help of integrins and cell adhesion molecules (CAMs) on the cell surface of the target cell and (2) inducing cell membrane damage by the release of pore-forming proteins (called perforins). These pores facilitate the unregulated entry of the pro-apoptotic protease granzyme, water, and salts. The cytolytic T cell protects itself by a membrane protein, protectin, that inactivates perforin, blocking its insertion into the cytolytic T cell membrane.
Cytolytic T cells can also destroy target cells by the Fas-Fas ligand mechanism seen during apoptosis (see Chapter 3, Cell Signaling). When the cytolytic T cell receptor recognizes an antigen on the surface of a target cell, Fas ligand is produced in the cytolytic T cell. The interaction of Fas ligand with the trimerized Fas receptor on the target cell surface (see Figure 10-9) triggers the apoptotic cascade by activation of procaspases into caspases that determine cell death.
Regulatory, suppressors, and effector T cells
Suppressor T cells include Th1 and Th2 cells, two subsets of T cells. Suppressor T cells acting on helper T cells to moderate or inhibit their activities, also modulate the differentiation of B cells into plasma cells. Suppressor T cells produce different cytokines with distinct functions. Th1 cells produce interferon-γ, whereas Th2 cells produce IL-4 and IL-13. Interferon-γ, produced by Th1 cells, stimulates the differentiation of Th1 cells but suppresses the proliferation of Th2 cells. Furthermore, TH2-derived IL-4 suppresses the activation of Th1 cells.
Natural killer cells destroy virus-infected cells and tumor cells, but this activity does not depend on antigen activation. Natural killer cells do not belong to the T or B cell types (they do not express TCR). They have CD56 receptors as well as inhibitory and activating receptors interacting respectively with class I MHC and activating ligand of normal cells. Target cells lacking class I MHC activate the destructive function of natural killer cells. The mechanism by which natural killer cells destroy target cells is described in Figure 10-10.
Clinical significance: Acquired immunodeficiency syndrome
Figure 10-11 presents a summary of the cellular events associated with HIV infection. Box 10-F summarizes the steps of the HIV reproductive cycle. A relevant event of HIV infection is the destruction of CD4+ helper T cells responsible for the initiation of immune responses, leading to the elimination of HIV infection. Cytolytic T cells (that attach to virus-infected cells) and B cells (that give rise to antibody-producing plasma cells) represent an adaptive response to HIV infection. Antibodies to HIV antigens are detected within 6 to 9 weeks after infection.
Box 10-F HIV reproductive cycle
Clinical significance: Allergy
Allergy refers to immune responses characterized by the participation of IgE bound to a special receptor, designated FcRI. When an antigen or allergen binds to two adjacent IgE molecules, it induces aggregation of the IgE molecules and associated Fc
RI receptors. This event results in a signaling cascade that leads to the release of mediators and cytokines (Figure 10-12).
Complement system
The main function of the complement system is to enable the direct destruction of pathogens or target cells by phagocytes (macrophages and neutrophils) by a mechanism known as opsonization (Greek opsonein, to buy provisions) by producing proteolytic enzyme complexes (Figure 10-13).
The complement system has the following specific characteristics important to remember:
LYMPH NODES
Structure of a lymph node
A lymph node is surrounded by a capsule, and the parenchyma is divided into a cortex and a medulla (Figure 10-14). The capsule consists of dense irregular connective tissue surrounded by adipose tissue. The capsule at the convex surface of the lymph node is pierced by numerous afferent lymphatic vessels. Afferent lymphatic vessels have valves to prevent the reflux of lymph entering a lymph node.
A lymphoid follicle (Figure 10-15) consists of a mantle (facing the cortex) and a germinal center containing mainly proliferating B cells or lymphoblasts, resident follicular dendritic cells (FDCs), migrating dendritic cells (see Box 10-F), macrophages, and supporting reticular cells, which produce reticular fibers (type III collagen). A primary lymphoid follicle lacks a mantle and germinal center. A secondary lymphoid follicle has a mantle and a germinal center. The mantle and germinal center develop in response to antigen stimulation.
Lymphatic sinuses are spaces lined by endothelial cells. They are located under the capsule (subcapsular sinus) and along trabeculae of connective tissue derived from the capsule and entering the cortex (paratrabecular sinus). Highly phagocytic macrophages are distributed along the subcapsular and paratrabecular sinuses to remove particulate matter present in the percolating lymph. Lymph entering the paratrabecular sinus through the subcapsular sinus percolates to the medullary sinuses and exits through a single efferent lymphatic vessel. Lymph in the subcapsular sinus can bypass the paratrabecular and medullary sinuses and exit through the efferent lymphatic vessel.
High endothelial venules (HEVs) (see Figure 10-14), located in the inner cortex, are the sites of entry of most B and T cells into the lymph node (by the lymphocyte homing mechanism).
The medulla is surrounded by the cortex, except at the region of the hilum (see Figure 10-14). The hilum is a concave surface of the lymph node where efferent lymphatic vessels and a single vein leave and an artery enters the lymph node.
The medulla contains two major components:
Clinical significance: Lymphadenitis and lymphomas
In Chapter 12, Cardiovascular System, we discuss that the interstitial fluid, representing plasma filtrate, is transported into blind sacs corresponding to lymphatic capillaries. This interstitial fluid—entering the lymphatic capillaries as lymph—flows into collecting lymphatic vessels becoming afferents to regional lymph nodes (see Box 10-G). Lymph nodes are linked in series by the lymphatic vessels in such a way that the efferent lymphatic vessel of a lymph node becomes the afferent lymphatic vessel of a downstream lymph node in the chain.
Box 10-G Lymph flow and dendritic cell migration
Soluble and particulate antigens drained with the interstitial fluid, as well as antigen-bearing dendritic cells in the skin (Langerhans cells; see Chapter 11, Integumentary System), enter the lymphatic vessels and are transported to lymph nodes. Antigen-bearing dendritic cells enter the CD4+ helper T cell–rich inner cortex. Soluble and particulate antigens are detected in the percolating lymph by resident macrophages and dendritic cells strategically located along the subcapsular and paratrabecular sinuses.
Lymphomas are tumors of the lymphoid tissue in the form of tissue masses. The designation lymphocytic leukemia is used for lymphoid tumors involving the bone marrow. Most of the lymphomas are of B cell origin (80%); the remainder are of T cell origin. Lymphomas include Hodgkin’s lymphoma (Figure 10-16) and non-Hodgkin’s lymphomas. They are clinically characterized by nontender enlargement of localized or generalized lymph nodes (nodal disease). The Hodgkin-Reed-Sternberg cell, found in classic Hodgkin’s lymphoma, is a large multinucleated or multilobulated tumor cell of B cell origin surrounded by T cells, eosinophils, plasma cells, and macrophages (mixed celullarity). Another group in the lymphoma category includes the plasma cell tumors, consisting of plasma cells, the terminally differentiated B cells. Plasma cell tumors (multiple myeloma) originate in bone marrow and cause bone destruction with pain due to fractures.
THYMUS
Development of the thymus
A brief review of the development of the thymus facilitates an understanding of the structure and function of this lymphoid organ. The mesenchyme from the pharyngeal arch gives rise to the capsule, trabeculae, and vessels of the thymus (Figure 10-17). The thymic epithelial rudiment attracts bone marrow–derived thymocyte precursors, dendritic cells, and macrophages required for normal thymic function.
During fetal life, the thymus contains lymphocytes derived from the liver. T cell progenitors formed in the bone marrow during hematopoiesis enter the thymus as immature thymocytes and mature to become immunocompetent T cells (predominantly CD4+ or CD8+), which are then carried by the blood into lymph nodes, spleen, and other lymphatic tissues (Figure 10-18).
The thymus in humans is fully developed before birth. The production of T cells is significant before puberty. After puberty, the thymus begins to involute and the production of T cells in the adult decreases. The progenies of T cells become established, and immunity is maintained without the need to produce new T cells.
Box 10-H Aire gene and autoimmunity
Thymic cortical epithelial cells are involved in the clonal selection of T cells. Medullary epithelial cells are involved in the clonal deletion of potentially au to reactive T cells.
Structure of the thymus
The thymus consists of two lobes subdivided into incomplete lobules, each separated into an outer cortex and a central medulla (Figure 10-19). A connective tissue capsule with small arterioles surrounds the lobules. The capsule projects septa or trabeculae into the organ. Blood vessels (trabecular arterioles and venules) within the trabeculae gain access to the thymic epithelial stroma (Figure 10-20).
The cortex contains thymic epithelial cells forming a three-dimensional network supported by collagen fibers. Thymic epithelial cells, linked to each other by desmosomes, surround capillaries. A dual basal lamina is present in the space between epithelial cells and capillaries. One basal lamina is produced by the cortical thymic epithelial cells. The other basal lamina is of endothelial cell origin. Macrophages may also be present in proximity (Figure 10-21).
Thymic cortical epithelial cells, basal laminae, and endothelial cells form the functional blood-thymus barrier (see Figure 10-21). Macrophages adjacent to the capillaries ensure that antigens escaping from blood vessels into the thymus do not react with developing T cells in the cortex, thus preventing the risk of an autoimmune reaction.
Most T cell development takes place in the cortex. In the outer area of the cortex adjacent to the capsule, double-negative thymocytes proliferate and begin the process of gene rearrangement leading to the expression of the pre-TCR along with coreceptors CD4 and CD8 (see Figures 10-7 and 10-20).
T cells that recognize self-MHC molecules but not self-antigens are allowed to mature by positive selection. T cells unable to recognize MHC molecules are not selected and are eliminated by programmed cell death, or apoptosis (see Chapter 3, Cell Signaling).
About 95% of the developing T cells die within the cortex of the thymus without ever maturing. Double-positive T cells undergo apoptosis within three days in the absence of a surviving signal; positive signals enable the progression to single-positive. Within 1 week, single-positive cells will be eliminated by apoptosis unless they receive a positive signal for survival and export to the periphery.
The medulla of one lobule is continuous with the medulla of an adjacent lobule. The medulla displays few almost mature T cells (single-positive) migrating from the cortex. Maturation of T cells is completed in the medulla, and functional T cells enter postcapillary venules in the corticomedullary junction to exit the thymus toward the peripheral lymphoid organs (see Figure 10-20).
Thymic epithelial cells populate the medulla, many of them forming Hassall’s corpuscles. Hassall’s corpuscles are sites where thymic epithelial cells accumulate and form onion-like layers (see Figure 10-20). Hassall’s corpuscles produce cytokine thymic stromal lymphopoietin, which stimulates thymic dendritic cells to complete the maturation of single-positive T cells to optimize negative selection and ensure tolerance.
Note that the blood-thymus barrier is not present in the medulla and that Hassall’s corpuscles can be seen only in the medulla.
SPLEEN
The spleen has two major components with distinct functions (Figure 10-22): the white pulp and the red pulp.
The white pulp is the immune component of the spleen. The cell components of the white pulp are similar to those of the lymph node, except that antigens enter the spleen from the blood rather than from the lymph.
Vascularization of the spleen
Trabeculae derived from the capsule carry blood vessels (trabecular arteries and veins) and nerves to and from the splenic red pulp (Figure 10-22). A brief review of vascularization of the spleen, which is similar to that of many organs with a significant blood supply such as the kidneys and lungs, provides a useful background for understanding the function and structure of this organ.
White pulp
The white pulp includes (see Figure 10-19) (1) the central artery or arteriole surrounded by a sheath of T cells (PALS) and (2) the lymphatic nodules, consisting of B cells. Antigen-presenting cells and macrophages are also present in the white pulp.
There is a marginal sinus zone between the red and white pulps that receives radial arterioles from the central artery or arteriole (Figures 10-23 and 10-24). This marginal sinus zone drains into small sinusoids located on the outer portion of the marginal zone. At the marginal zone, blood contacts the splenic parenchyma, which contains phagocytic macrophages and antigen-presenting cells. T and B cells enter the spleen and become segregated in their specific splenic location.
Red pulp
The red pulp contains an interconnected network of splenic sinusoids lined by elongated endothelial cells separated by narrow slits. Splenic cords, also known as the cords of Billroth, separate splenic sinusoids (see Figure 10-23; Figure 10-25).
Splenic sinusoids are discontinuous vascular spaces lined by rib-shaped endothelial cells oriented in parallel along the long axis of the sinusoids (see Figure 10-25). Junctional complexes can be found at the tapering ends of the endothelial cells.
Each splenic sinusoid is covered by a discontinuous basal lamina oriented like barrel hoops around the endothelial cells (see Figure 10-25). Adjacent hoops are cross-linked by strands of basal lamina material. In addition, a network of loose reticular fibers also encircles the splenic sinusoids. Consequently, blood cells have an unobstructed access to the sinusoids through the narrow slits between the fusiform endothelial cells and the loose basal lamina–reticular fiber network.
Two types of blood circulations have been described in the red pulp (see Figure 10-23): (1) a closed circulation, in which arterial vessels connect directly to splenic sinusoids; and (2) an open circulation, characterized by blood vessels opening directly into the red pulp spaces, with the blood flowing through these spaces and then entering through the interendothelial cell slits of the splenic sinusoids.
Clinical significance: Sickle cell anemia
Sickle cell anemia is discussed briefly in Chapter 6, Blood and Hematopoiesis, within the context of the structure of the red blood cell. Here, we focus on the fate of irreversibly sickled red blood cells when they travel through the narrow passages of the red pulp. We also consider the function of macrophages associated with the splenic sinuses in the disposal of destroyed sickle cells.
When the oxygen tension decreases, sickle cells show preferential adhesion to postcapillary venules followed by trapping of irreversibly sickled cells and retrograde obstruction of the blood vessel (Figure 10-26).
An increased destruction of sickle cells leads to anemia and to an increase in the formation of bilirubin from the released hemoglobin (chronic hyperbilirubinemia). The occlusion of splenic sinuses by sickle cells is associated with splenomegaly (enlargement of the spleen), disrupted bacterial clearance function of the spleen in cases of bacteremia, and painful crises in the affected region. Similar vascular occlusions can also occur in the kidneys, liver, bones, and retinas.
Clinical significance: Adoptive cellular immunotherapy
Two procedures have been used (Figure 10-27):
Immune-Lymphatic System