Fig. 2.1
Electron micrographs of glomerular capillary segments from sections of rat kidney following infusion in vivo with either Hpx (a) or heat-inactivated Hpx (HI-Hpx) (b). The tissue samples were taken 2 h after infusion and stained for anionic sites using polyethyleneimine (1.8 kD) as a cationic marker. It can be seen that following contact with Hpx (a), fusion of epithelial foot processes is present (arrows), in contrast to the rat kidney infused with HI-Hpx (b). Also, relative reduction of the amount of anionic sites along the LRI as compared with the lamina rara externa can be observed in Hpx-infused kidneys (a) vs. HI-Hpx (b). C capillary lumen, U urinary space. Final magnification ×47,619 [30]
The effects of active Hpx are observed on human podocyte and glomerular endothelial cells (GEnC) monolayers, and an additional mechanism for proteinuria is reported [31]. After Hpx treatment, both podocytes and GEnC demonstrated a reduction in the expression of glycocalyx, which is composed of glycoproteins including proteoglycans and which coats the luminal surface of the glomerular capillaries. In GEnC, the disruption of glycocalyx was associated with an increase in the flux of albumin, without any changes in the morphology of GEnC monolayers. The albumin-restrictive properties of glycocalyx are consistent as reported that it contributes to the barrier to flux of albumin across the cell layer [32].
As foot process effacement has been demonstrated in Fig. 2.1a after infusion of Hpx, changes observed in the filamentous actin cytoskeleton of human podocytes are reported. Wild-type (WT) podocytes showed dramatic reorganization of actin with loss of stress fibers and the development of membrane ruffles and cytoplasmic aggregates (Fig. 2.2). The effects of Hpx on actin were reversible within 4 h, and this is in keeping with the time course of proteinuria seen after Hpx infusion in rats [30]. This reversibility could be explained by receptor-binding dynamics or by the physiologic stability of Hpx.
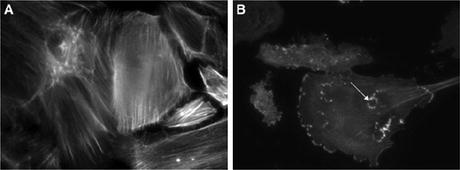
Fig. 2.2
(a) Wild-type (WT) podocytes exhibited actin in stress fibers in normal culture conditions and in serum-free medium. (b) By contrast, WT podocytes treated with Hpx (0.05 mg/ml for 30 min) showed dramatic reorganization of actin with loss of stress fibers and peripheral ruffles and the development of cytoplasmic aggregates. In some cells, these aggregates resembled podosomes (arrow). Images are representative of n = 6 independent experiments. Final magnification ×400 [31]
On the other hand, nephrin-deficient (ND) podocytes did not show actin reorganization after Hpx treatment, indicating that the Hpx effect on actin was dependent on the expression of nephrin. This finding was further supported by nephrin siRNA knockdown experiments in WT podocytes, where there was a significant reduction in actin reorganization after Hpx treatment. Furthermore, reconstitution of nephrin in ND podocytes was associated with changes in the actin cytoskeleton after Hpx, and this was comparable to the changes in WT podocytes (Fig. 2.3). These observations firmly establish a link between Hpx treatment, nephrin expression, and actin reorganization in podocytes. Therefore, it is hypothesized that nephrin plays a key role in the downstream relay of intracellular signaling leading to actin reorganization in podocytes in MCNS.
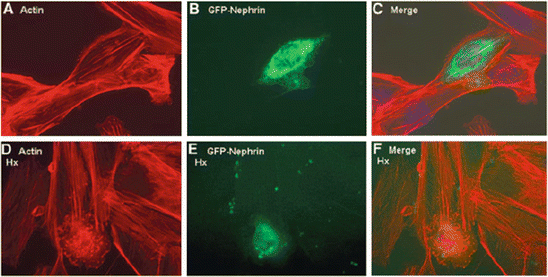
Fig. 2.3
Nephrin-deficient (ND) podocytes were reconstituted with green fluorescent protein (GFP)-tagged nephrin by microinjection (a through c). Single microinjected cell expressing GFP-nephrin (b) demonstrated actin stress fibers in serum-free medium, similar to uninjected cells surrounding it (a and merged in c). (d through f) When these cells were treated with Hpx (0.05–1.00 mg/ml for 30 min), only the GFP-nephrin-expressing cell (e) demonstrated actin reorganization (d and merged in f). Images are illustrative of n = 3 experiments each performed in triplicate. Final magnification ×400 [31]
The mechanism of Hpx’s effects on the actin cytoskeleton was explored by studying key cytoskeletal signaling pathways [31]. WT and ND podocytes showed differences in activation of key cytoskeletal signaling pathways after Hpx treatment. RhoA is a small GTPase, and, when activated, it is a molecular switch that can lead to actin reorganization. In WT podocytes, there was minimal basal activation of RhoA, and this increased after Hpx. In association with this, there was observed actin reorganization. By contrast, ND cells had no change in RhoA activation after Hpx. In association with this, ND podocytes had no associated actin reorganization. These findings suggest that nephrin is required to regulate RhoA activation in response to Hpx. Protein kinase B (PKB) is central to many intracellular signaling pathways, and it has been associated with actin reorganization [33] and nephrin signaling [34]. In WT and ND podocytes, there was minimal additional phosphorylation of PKB at T308 after Hpx treatment; however, phosphorylation at S473 was upregulated considerably by Hpx in WT cells compared with ND cells. This suggests that the phosphorylation of PKB after Hpx stimulation occurs at the S473 site via nephrin. The previously reported nephrin-associated activation of PKB was also at S473 [34].
Thus, Hpx has been shown to affect every layer of the glomerular filtration barrier with its protease activity in histochemistry and in molecular cell biology findings, but the mechanisms of Hpx in causing MCNS still remain to be elucidated. For example, whether Hpx binds a cell surface receptor is unknown. The heme–Hpx complex is known to bind to a specific receptor low-density lipoprotein receptor-related protein 1 on hepatocytes, to allow heme transfer [35]; however, Hpx is not known to be a ligand for other receptors. Because it has serine protease activity, Hpx may act via the family of protease-activated receptors. Interestingly, more than 500 proteins have domains showing homology to the Hpx molecule, among which there are the matrix metalloproteinases. These proteins have been implicated in many disease processes, and recent studies investigated the possibility of a matrix metalloproteinase–protease-activated receptor 1 (PAR1) signaling axis [36]. It is recently reported that proteases present in nephrotic plasma obtained from patients with focal segmental glomerulosclerosis (FSGS) can activate PAR1, leading to the podocin-dependent phosphorylation of actin-associated protein vasodilator-stimulated phosphoprotein (VASP) in human podocyte suggesting a novel role for proteases and PARs in the pathogenesis of FSGS [37].
2.3.2 Hemopexin and Relapse of Pathophysiological Mechanisms in MCNS Patients
A question is raised about what the mechanism is in the onset or in the relapse of MCNS caused by active Hpx.
It has been suggested that various isoforms of Hpx exist and that in normal conditions circulating Hpx is inactive, but under certain circumstances Hpx becomes activated as a serine protease. Altered activity of plasma Hpx in MCNS in relapse is demonstrated [38]. A decreased mean titer of plasma Hpx is seen specifically in relapsed subjects as compared with remission [38], as reported in recent plasma and urine proteomic profiles analysis in childhood MCNS [39]. Increased Hpx protease activity exclusively in plasma from MCNS relapsed subjects is also indicated with different Western blot patterns compared to remission plasma and plasma from other proteinuric subjects with FSGS, membranoproliferative glomerulonephritis (MPGN), or IgA nephropathy or healthy control individuals. With respect to the Western blot patterns of plasma from subjects with MCNS in relapse, it appeared that 12 out of 18 subjects showed 80-kD bands as well as 65-kD bands, as detected by monoclonal anti-Hpx IgG, whereas only 65-kD bands are detected in remission samples. Control donors or patients with FSGS, MPGN, or IgA nephropathy showed various blot patterns. In terms of utility of Hpx as a biomarker, it could be further studied to distinguish the differences between relapse and remission or MCNS in relapse and FSGS, with careful clinical phenotyping of the FSGS patients.
Another interesting observation was that preincubation of podocytes in normal human plasma prevented actin reorganization after Hpx treatment. This indicates that factors in normal plasma act to protect podocytes [31]. In MCNS, there may be loss of such factors from the plasma, leaving podocytes exposed to the effects of activated Hpx. These plasma factors could be acting directly on podocytes to regulate the expression of receptors or to maintain the integrity of the slit diaphragm complex. Alternatively, these factors could be acting as direct inhibitors of active Hpx in a similar manner to other circulating proteases that have circulating inhibitors. In support of this theory, as previously indicated, the serine protease inhibitor reduced the effect of Hpx on the actin cytoskeleton in WT cells and on glomerular extracellular matrix molecules [27, 29, 31].
In contrast to inhibitory factors for active Hpx in normal plasma, there is a report of activation mechanism of Hpx in endothelial and mesangial cells in paracrine manner in glomeruli. Human mesangial cells stimulated with tumor necrosis factor-alpha (TNF-α) release Hpx in vitro in a corticosteroid-dependent manner [14]. The enzymatic isoform of Hpx could be inactivated by extracellular nucleotides like ADP or ATP. The protease activity of inactivated Hpx could be restored by treatment with soluble apyrase yielding the enzymatic active form of this molecule [40]. Inflammatory agents like lipopolysaccharide or TNF-α are able to upregulate ecto-ADPase of endothelial or mesangial cells in vitro, and secondly, inactive isoform of Hpx can be converted to active isoform by ecto-ADPase present along the surface of these cells. Prednisolone is able to downregulate ectoapyrase in stimulated endothelial or mesangial cells, which may potentially inhibit the conversion of Hpx to its active isoform [41]. This hypothesis may explain the clinical feature of onset or relapse of MCNS triggered by viral infection or as it has been indicated in terms of immune cell disturbance in MCNS that TNF-α synthesis in peripheral mononuclear cells from relapse is increased [42] and that the promoter region of TNF-α in naïve T-helper cells from relapse has a significant reduction in DNA methylation compared to that from remission in the same patients indicating predisposition of TNF-α synthesis in relapse (personal communication).
2.4 Conclusion
The active isoform of Hpx shows serine protease activity, though it is still needed to clarify whether Hpx itself has serine protease activity or Hpx-like domain in other proteins shows this activity. The isoform has been indicated to induce enhanced glomerular permeability for albumin, effacement of epithelial foot processes, a reduction of a cationic marker in the LRI of glomerular basement membrane, reduction of glycocalyx of the surface of GEnC, and loss of actin stress fibers, which is dependent on nephrin expression and followed by RhoA activation and PKB phosphorylation at S473 in the downstream intracellular signaling pathway after Hpx treatment. These effects of Hpx on the glomerular filtration barrier were reversible and blocked by healthy plasma. Potential future therapy for MCNS could target the receptors or signaling pathways involved or focus on inhibitory factors for active Hpx. The possibility of proteases is raised as a circulating factor causing MCNS, with the potential intermediate mechanism of inflammatory cytokines such as TNF-α activating such factors during acute infections or the resultant immune activation. This suggests future directions to elucidate the full pathogenesis of MCNS from the typical clinical course of relapse to therapeutic response.
References
1.
3.
Takahashi N, Takahashi Y, Putnam FW. Complete amino acid sequence of human hemopexin, the heme-binding protein of serum. Proc Natl Acad Sci U S A. 1985;82:73–7.PubMedCentralCrossRefPubMed
4.
Smith A. Role of redox-reactive metals in the regulation of the metallothionein and heme oxygenase genes by heme and hemopexin. In: Ferreira GC, Moura JJG, Franco R, editors. Iron metabolism. Weinheim: Wiley-VCH; 1999. p. 65–92.CrossRef
5.
6.
7.
8.
Thorbecke GJ, Liem HH, Knight S, Cox K, Muller-Eberhard U. Sites of formation of the serum proteins transferrin and hemopexin. J Clin Invest. 1973;52:725–31.PubMedCentralCrossRefPubMed
9.
Immenschuh S, Nagae Y, Satoh H, Baumann H, Muller-Eberhard U. The rat and human hemopexin genes contain an identical interleukin-6 response element that is not a target of CAAT enhancer-binding protein isoforms. J Biol Chem. 1994;269:12654–61.PubMed
10.
11.
Hunt RC, Hunt DM, Gaur N, Smith A. Hemopexin in the human retina: protection of the retina against heme-mediated toxicity. J Cell Physiol. 1996;168:71–80.PubMed
12.
Swerts JP, Soula C, Sagot Y, Guinaudy MJ, Guillemot JC, Ferrara P, et al. Hemopexin is synthesized in peripheral nerves but not in central nervous system and accumulates after axotomy. J Biol Chem. 1992;267:10596–600.PubMed
13.
14.
15.
Smith A, Morgan WT. Haem transport to the liver by haemopexin. Receptor-mediated uptake with recycling of the protein. Biochem J. 1979;182:47–54.PubMedCentralCrossRefPubMed
16.
Smith A, Morgan WT. Haemopexin-mediated transport of heme into isolated rat hepatocytes. J Biol Chem. 1981;256:10902–9.PubMed
17.
Smith A, Hunt RC. Hemopexin joins transferrin as representative members of a distinct class of receptor-mediated endocytic transport systems. Eur J Cell Biol. 1990;53:234–45.PubMed
18.
Thomas L. Haptoglobin hemopexin. In: Thomas L, editor. Clinical laboratory diagnostics. Frankfurt: TH-Books; 1998. p. 663–7.
19.
Weeke B, Jarnum S. Serum concentration of 19 serum proteins in Crohn’s disease and ulcerative colitis. Gut. 1971;12:297–302.PubMedCentralCrossRefPubMed
20.
Johnson AM. Amino acids, peptides and proteins. In: Burtis CA, Ashwood EER, Bruns DE, editors. Tietz textbook of clinical chemistry and molecular diagnostics. St. Louis: Elsevier, Saunders Company; 2006. p. 533–95.
21.
22.
23.
Cheung PK, Boes A, Dijkhuis FWJ, Klok PA, Bakker WW. Enhanced glomerular permeability and minimal change disease like alterations of the rat kidney induced by a vasoactive human plasma factor. Kidney Int. 1995;47:1218.
24.
25.
26.
Vernier RL. Primary (idiopathic) nephrotic syndrome. In: Holliday MA, Barratt TM, Vernier RL, editors. Paediatric nephrology. Baltimore: Williams and Wilkins; 1987. p. 445–56.
27.
Cheung PK, Stulp B, Immenschuh S, Borghuis T, Baller JF, Bakker WW. Is 100KF an isoform of hemopexin? Immunochemical characterization of the vasoactive plasma factor 100KF. J Am Soc Nephrol. 1999;10:1700–8.PubMed
28.
Mauk MR, Smith A, Mauk AG. An alternative view of the proposed alternative activities of hemopexin. Protein Sci. 2011;20:791–805.PubMedCentralCrossRefPubMed
29.
30.
31.
Lennon R, Singh A, Welsh GI, Coward RJ, Satchell S, Ni L, et al. Hemopexin induces nephrin-dependent reorganization of the actin cytoskeleton in podocytes. J Am Soc Nephrol. 2008;19:2140–9.PubMedCentralCrossRefPubMed
32.
33.
34.
Huber TB, Hartleben B, Kim J, Schmidts M, Schermer B, Keil A, et al. Nephrin and CD2AP associate with phosphoinositide 3-OH kinase and stimulate AKT-dependent signaling. Mol Cell Biol. 2003;23:4917–28.PubMedCentralCrossRefPubMed
35.
36.
37.
38.
39.
40.
Kapojos JJ, Poelstra K, Borghuis T, Banas B, Bakker WW. Regulation of plasma hemopexin activity by stimulated endothelial or mesangial cells. Nephron Physiol. 2004;96:1–10.CrossRef
41.
42.
Bakr A, Shokeir M, El-Chenawi F, El-Husseni F, Abdel-Rahman A, El-Ashry R. Tumor necrosis factor-alpha production from mononuclear cells in nephrotic syndrome. Pediatr Nephrol. 2003;18:516–20.PubMed