14 Hearing and Balance: The Eighth Cranial Nerve
The eighth nerve is the nerve of hearing and equilibrium. All of its receptive functions are accomplished by variations on a common theme; the different sensory information carried by different fibers in the nerve is simply the result of slight differences in the mechanical arrangement of receptors and accessory structures.
Auditory and Vestibular Receptor Cells Are Located in the Walls of the Membranous Labyrinth
Auditory and Vestibular Receptors Are Hair Cells
Hair cells, the characteristic receptor cells of the labyrinth, have a graduated array of specialized microvilli (stereocilia) and sometimes one true cilium (the kinocilium) on their apical surfaces. Each stereocilium is attached to its next tallest neighbor by a filamentous tip link protein, connected at one or both ends to a cation channel. The sensory hairs of the hair cells poke through the wall of the membranous labyrinth and are typically inserted into a mass of gelatinous material (Fig. 14-1). Movement of a hair bundle relative to the gelatinous material causes a depolarizing or a hyperpolarizing receptor potential, depending on the direction of deflection. Deflecting the hair bundle toward the tallest stereocilia stretches the tip links, opens the cation channels, and depolarizes the hair cell; deflecting in the opposite direction lets the tip links relax, and some channels that were open at rest close. This in turn causes an increase or a decrease in the release of an excitatory transmitter (probably glutamate), and a consequent increase or decrease in the firing rate of any eighth nerve fiber that innervates the hair cell (Fig. 14-2). The way in which the gelatinous material is arranged within the labyrinth plays a major role in determining the kind of mechanical stimulus to which a particular region of the labyrinth responds best.
The Cochlear Division of the Eighth Nerve Conveys Information about Sound
The Cochlea Is the Auditory Part of the Labyrinth
The cochlear duct is stretched as a partition across the cochlear part of the bony labyrinth. The partition is complete except for a small hole at the apex of the cochlea (the helicotrema) at which two otherwise separate perilymphatic spaces communicate with each other. Therefore when the stapes moves inward and outward, part of the resulting perilymph movement causes a traveling wave of deformation that moves along the cochlear duct. The deformation reaches a maximum amplitude at a site that depends on the frequency of the stapes vibration (Fig. 14-3); portions of the cochlear duct closer to the oval window are more sensitive to higher frequencies, and portions closer to the helicotrema are more sensitive to lower frequencies. This is, at least to a great extent, the result of gradual changes in the width and mechanical properties of the basilar membrane, which forms one wall of the cochlear duct. Cochlear hair cells are located in the organ of Corti (on the basilar membrane), with their sensory hairs embedded in the gelatinous tectorial membrane (Fig. 14-4). Deformation of the cochlear duct causes differential movement of the basilar and tectorial membranes and this deflects the sensory hairs, which in turn causes either a depolarizing or a hyperpolarizing receptor potential in the hair cells (depending on the direction of deflection).
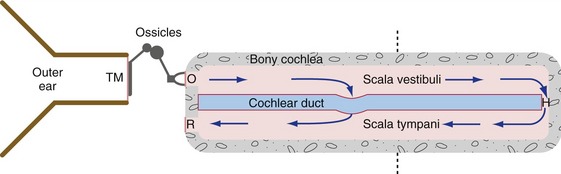
Figure 14-3 Outer, middle, and inner ear, shown schematically as though the cochlea had been uncoiled. Vibrations transmitted through the tympanic membrane (TM), middle ear ossicles, and oval window (O) reach the perilymph of the inner ear. Very low frequencies and static pressure changes move perilymph through the helicotrema (H), but audible frequencies deform the cochlear duct. The dashed line indicates the plane of section in Fig. 14-4. R, Round window membrane.
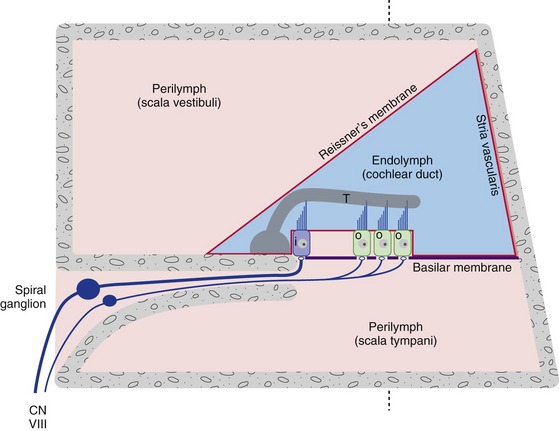
Figure 14-4 A schematic cross section through one turn of the cochlea, showing the organ of Corti with its inner (i) and outer (o) hair cells. The tallest stereocilia of at least the outer hair cells are inserted in the gelatinous tectorial membrane (T). All three walls of the cochlear duct contain a diffusion barrier separating endolymph and perilymph. Reissner’s membrane does little more than this, but the stria vascularis is specialized as a secretory epithelium that produces endolymph. The perilymph of scala vestibuli (open to the vestibule) is continuous with that of scala tympani (ends at the round window, or secondary tympanic membrane) at the helicotrema. The dashed line indicates the plane of section in Fig. 14-3.
Auditory Information Is Distributed Bilaterally in the CNS
We use our ears not only to identify sounds, but also to localize them in space. This localization is achieved by comparing time and intensity differences between the sounds arriving at our two ears, and the comparison begins early in the CNS. Cochlear nerve fibers end ipsilaterally in the cochlear nuclei at the pontomedullary junction. The cochlear nuclei then project bilaterally in the brainstem, so at all levels rostral to the cochlear nuclei each ear is represented bilaterally (Fig. 14-5) and unilateral damage does not cause deafness of either ear. Rostral to the cochlear nuclei, the auditory pathway on each side is concerned not so much with one ear as with information from both ears relevant to the contralateral half of the auditory world.
Successively more rostral stages in the auditory pathway (THB6 Figure 14-18, p. 359) include the superior olivary nucleus (crossing fibers reach it through the trapezoid body); inferior colliculus (by way of the lateral lemniscus); medial geniculate nucleus of the thalamus (by way of the brachium of the inferior colliculus, or inferior brachium); and auditory cortex (transverse temporal gyri on the superior surface of the temporal lobe).
Efferents Control the Sensitivity of the Cochlea
Two small muscles—the stapedius, attached to the stapes, and the tensor tympani, attached to the malleus—affect middle ear function by tugging on ossicles and decreasing their ability to transmit vibrations. The tensor tympani is only activated by very loud sounds, and its function is unclear. However, the stapedius is activated bilaterally in response to loud sounds near the upper end of the physiological range of intensities. Stapedial contraction makes the tympanic membrane harder to vibrate, so more sound gets reflected from it. Hence measuring the amount of a test sound reflected from one tympanic membrane provides an indirect measure of stapedius contraction on that side. The circuitry of the acoustic (stapedius) reflex involves both the seventh and eighth nerves, as well some of their central connections (Fig. 14-6), so this forms the basis of a useful clinical test.
The Vestibular Division of the Eighth Nerve Conveys Information about Linear and Angular Acceleration of the Head
Receptors in the Utricle and Saccule Detect Linear Acceleration and Position of the Head
The utricle and saccule each contains a flattened patch of hair cells (a macula) overlain by a layer of gelatinous material containing crystals of calcium carbonate (hence called an otolithic membrane, or a “membrane full of ear stones”). The otolithic membrane is denser than endolymph and so tries to move through it in response to gravity or other linear accelerations (Fig. 14-7). This in turn deflects the hair bundles inserted into the otolithic membrane and elicits depolarizing or hyperpolarizing receptor potentials. The utricular macula is oriented in a mostly horizontal plane (i.e., stereocilia pointing toward the sky), the saccular macula in a mostly vertical plane. Hence, the utricle is most sensitive to tilts beginning from a head-upright position, the saccule to tilts beginning from a head-sideways position. In response to linear acceleration in its plane, an otolithic membrane lags behind because of inertia. As a result, the utricle also responds to acceleration in horizontal planes (front-back, side to side), and the saccule to acceleration in vertical planes (front-back, up-down).
Receptors in the Semicircular Ducts Detect Angular Acceleration of the Head
The semicircular ducts use a different mechanism. Each contains a dilatation called an ampulla, in which the hair cells reside as part of a ridge called a crista. The hair bundles are inserted into a gelatinous diaphragm called a cupula. Movement of the endolymph within a semicircular duct would therefore distort the cupula and deflect the hair bundles (see Fig. 14-7).
The most effective stimulus for causing such movement is angular acceleration in the plane of a given canal (like a wheel on an axle). For example, at the beginning of rotation the endolymph lags behind because of inertia and pushes the cupula in the opposite direction. After a few seconds the endolymph catches up, and at the end of rotation it continues to move, again because of inertia, and pushes the cupula in the direction of the previous rotation (Fig. 14-8). The cupula normally has the same density as endolymph, so gravity has no effect on it. Processes that change the relative densities of cupula and endolymph can make one or more semicircular ducts gravity-sensitive, so certain head positions can fool the brain into thinking there’s some angular acceleration taking place. Vertigo results.
There are three semicircular canals and ducts on each side, in three orthogonal planes (Fig. 14-9). Roughly speaking, one is horizontal, one extends anteriorly at 45° to the sagittal plane, and one extends posteriorly at 45° to the sagittal plane. Hence, angular acceleration in any plane can be detected by each set of semicircular ducts.
The Vestibular Nuclei Project to the Spinal Cord, Cerebellum, and Nuclei of Cranial Nerves III, IV, and VI
Outputs from the vestibular nuclei go mostly to places that make sense from a functional point of view (Fig. 14-10; see THB6 Figure 14-29, p. 369). There is a vestibular projection through the thalamus to the cerebral cortex (insula and parietal lobe), but for the most part vestibular projections influence posture and eye movements.
The Vestibular Nuclei Participate in the Vestibulo-ocular Reflex
We can keep our eyes pointed at something even if we’re moving around. That is, a head movement of 5° to the left can be automatically nulled by a conjugate eye movement of 5° to the right. For slow head movements with your eyes open, this is partly a visual tracking movement, but you can do it even in the dark, and you can do it while your head is moving faster than visual tracking movements can work. The additional basis for these compensatory eye movements is the vestibulo-ocular reflex, a simple three-neuron reflex arc (Fig. 14-11). Vestibular primary afferents project to the vestibular nuclei, which then project (mostly, but not entirely, through the MLF) to the nuclei of III, IV, and VI.
Nystagmus Can Be Physiological or Pathological
Consider in a little more detail what would happen at the beginning of rotation to your left in the dark. At first, the vestibulo-ocular reflex would move your eyes to the right, the appropriate movement to keep the direction in which your eyes are pointed from changing (i.e., to keep your gaze from shifting). However, there is obviously a limit to how far your eyes can rotate in their sockets, and if you continue to spin around these compensatory movements are periodically interrupted by fast “reset” movements in the opposite direction. The combined back-and-forth movement is called nystagmus, and it gets named for the direction of the fast component. Hence, at the beginning of rotation to the left there would be nystagmus to the left (“left-beating nystagmus”). If rotation is maintained in the dark, the vestibular stimulus loses its effectiveness (see Fig. 14-8) and the rotatory nystagmus ceases. Right after a rotation ends, the direction of cupula deflection is the opposite of that at the beginning of rotation (see Fig. 14-8), and so there is postrotatory nystagmus in the opposite direction.
Position Sense Is Mediated by the Vestibular, Proprioceptive, and Visual Systems Acting Together
Information from these three systems needs to be integrated at an early stage. As one example, the semicircular canals can only signal maintained rotation for a little while, but the postural adjustments need to be maintained or you fall down. As a second example, if you were resting your head on your desk and your chair started to slide out, you’d probably make postural adjustments even though there had been no vestibular input. This integration starts to happen at the level of the vestibular nuclei, which up until now we have pretended received only vestibular and cerebellar inputs. In fact, the same cells receive somatosensory and visual inputs. In the first example, visual inputs to the vestibular nuclei cause continued nystagmus (called optokinetic nystagmus in this case because it is maintained by moving visual stimuli) and postural adjustments just as the original vestibular signal did. In the second example, the neck-vestibular mismatch would cause a vestibulospinal output.