Chapter 19 Future Considerations
Historical aspects
The field of ophthalmic ultrasonography essentially began in 1938 with the study of high intensity ultrasound effects on the eye by Zeiss.1,2 However, it was not until 1956 that the first ultrasonograph was published by Mundt and Hughes.3 The concept of a standardized ophthalmic ultrasonograph or standardized echography examination using A-mode was first introduced by Ossoinig in 1965 at the Eye Department of the University of Vienna.4 The following year, the International Society for Diagnostic Ophthalmic Ultrasound was founded.
Although B-mode ultrasound of the eye was first performed in 1958, it is the invention of the contact B-scan in 1972 that facilitated routine ophthalmic evaluation. The development of duplex Doppler ultrasound machines in the 1980s prompted diagnostic ophthalmic applications of this modality in the early 1990s.2 The availability of high frequency transducers led to the concept of ultrasound biomicroscopy, which was introduced by Pavlin in 1991.5 This and subsequent developments in ultrasound technology have greatly advanced the role of ophthalmic imaging in recent years. Some of the recent innovations and future considerations are discussed in the following sections.
High-frequency ultrasound and biomicroscopy
The use of micromachined ultrasound transducers has led to the development of clinically applicable high frequency transducers available for ophthalmologic ultrasonography in order to resolve small structures. The choroid, retina, and sclera can be readily distinguished at 20 MHz.6 Thus, high frequency ultrasound is superior to computerized tomography (CT) or magnetic resonance imaging (MRI) for detecting lesions that are 2 mm or less in thickness.7 While higher frequencies improve spatial resolution, there is a trade-off with depth of penetration. Nevertheless, probes with frequencies of 50 MHz and higher have been designed for ultrasound biomicroscopy.5 This technique generally uses B-mode ultrasonography with axial resolution in the order of 50 µm. The attenuation of the high-frequency ultrasound waves in biomicroscopy limits imaging to a depth of about 5 mm (Chapter 4).8
Nanotechnology applications in micromachining technologies enable fabrication of electrostatically driven membranes in the nanometer scale.9 Surface micromachined, capacitive ultrasonic transducers have been fabricated using complementary metal-oxide semiconductor (CMOS)-compatible processes. Novel transducer materials, such as lithium niobate and sol–gel composites, are capable of generating frequencies higher than 100 MHz.10
Doppler ultrasound
Doppler ultrasound is a technique that is used to evaluate blood flow, which can be depicted in color and in spectral tracings. Advances in Doppler ultrasound instrumentation permit detection of the slow-flow blood velocities in the ophthalmic artery and its branches, including the central retinal artery, the posterior ciliary artery, the lacrimal artery, as well as the superior ophthalmic vein, the vortex vein, and the central retinal vein (Chapter 5).11,12 Furthermore, Doppler spectral analysis can provide quantitative evaluation of blood flow velocity in these vessels. Thus, Doppler ultrasound plays a role in characterizing orbital tumors, carotid–cavernous sinus fistulas, central retinal artery occlusion, central retinal vein occlusion, giant cell arteritis, diabetic retinopathy, and ocular tumors (Figure 19.1).13 Power Doppler is superior to color Doppler technique for evaluation of the anatomy of orbital arteries and veins. Although sensitive to slow flow, the use of power Doppler in ophthalmic imaging is often limited due to artifacts.
Harmonic and superharmonic ultrasound
Harmonic imaging is based on the nonlinear interaction of the emitted ultrasound with the tissues, resulting in higher frequencies that return to the probe. This technique decreases artifacts and improves axial and lateral resolution. Imaging of first harmonics is widely used for many ultrasound applications. Superharmonic imaging is similar, but technically more challenging. Superharmonic imaging captures ultrasound beyond the second harmonic, yielding even greater contrast-to-tissue ratio. Detection of the transmission frequency up to the fifth harmonic is feasible, but necessitates a bandwidth greater than 130%. Phased array transducers with a wide dynamic range have been developed for this purpose. These transducers contain two different types of elements assembled in an interleaved pattern. The elements operate independently at different frequencies, allowing for separate transmission and reception modes.13–16 High-frequency contrast harmonic imaging of ophthalmic tumor perfusion has been reported (Figure 19.2).13–16
Contrast-enhanced ultrasound
Contrast-enhanced ultrasound (CEUS) is a technique that can be performed in real time following intravenous injection of microsphere or microbubble ultrasound contrast agents (UCA). Most UCA are composed of fluorocarbon or air and are stabilized by protein, lipid, or galactose-based substances. The half-life of UCA is several minutes. The imaging properties of UCA relate to the nonlinear interaction with the ultrasound waves, analogous to harmonic imaging (Chapter 5).
As compared to CT and MRI, CEUS is cost-effective, portable, produces no ionizing radiation, has no nephrotoxicity, and, most importantly, can provide comparable diagnostic information. Current applications of CEUS are for imaging visceral organs, abdominal aorta, genitourinary system, and breasts when standard ultrasound is inconclusive. In the realm of ophthalmic ultrasonography, UCA has been reported to slightly improve the detection of small vessels in uveal melanoma and helps differentiate a solid tumor from subretinal hemorrhage or effusion.17 However, CEUS does not distinguish normal vessels from tumoral vessels. CEUS has been combined with Doppler ultrasound to improve differentiation of retinal detachment from vitreous membrane (Figure 19.3).18
Compound imaging
Spatial compound ultrasound imaging is a technique that uses electronic beam steering to acquire several overlapping scans of an object from different view angles (Figure 19.4).19,20 Each single-angle scan is then averaged to create a multiangle image. This can be performed in real-time. The advantage of compound imaging over conventional ultrasound is reduction of speckle and clutter, resulting in better defined tissue boundaries. This technique is particularly well-suited for imaging rounded structures, such as the globe.
Three- and four-dimensional ultrasound
3D and 4D ultrasound provide additional diagnostic information for evaluating the orbit.21,22 For example, these modalities enable prenatal diagnosis of anophthalmia when the fetal head position is unfavorable on 2D ultrasound (Chapter 14) (Figure 19.5). The reverse face view function is particularly helpful. 3D ultrasound also has applications beyond the prenatal period. This technology can readily depict retinoblastoma and associated features, such as retinal detachment, intratumoral calcifications, and orbital shadowing.22 Oblique and coronal views of the tumor and optic nerve can be retrospectively derived from 3D ultrasound data and are useful for analyzing intraneural spread of tumor. 3D ultrasound can also be used for ocular and tumor-volume analysis.23
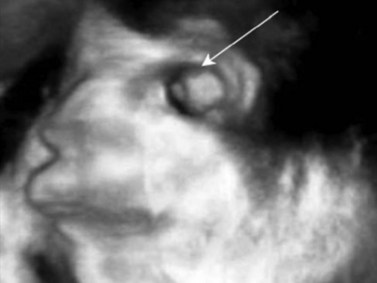
Figure 19.5 3D fetal ultrasound shows absence of the globe (arrow), compatible with anopthalmia.
Reproduced with permission from: Wong HS, Parker S, Tait J, Pringle KC. Antenatal diagnosis of anophthalmia by three-dimensional ultrasound: a novel application of the reverse face view. Ultrasound Obstet Gynecol 2008; 32(1):103–105.22
C-scan ultrasound
C-scan images are produced by sampling the ultrasound signal amplitude at fixed time intervals while the interrogating sensor is scanned over a surface (Figure 19.6) Coronal C-scan ultrasound is particularly useful for measuring the optic nerve and evaluating optic nerve sheath meningiomas and retinoblastoma invasion of the optic nerve.24–26
Ultrasound elastography
Elastography or elasticity imaging is a technique used to map tissue stiffness. Three main types of ultrasound elastography can be implemented: Compression strain imaging; external vibrations; acoustic radiation force or shear wave propagation through tissue using the ultrasound waves to determine the elastic modulus.27
Although currently under investigation, ultrasound elastography has demonstrated promising clinical applications for evaluating thyroid, breast, lymph nodes, and prostate lesions. 27,28 Indeed, the greater stiffness of malignant tissues with respect to benign or normal tissues can be differentiated with this modality (Figure 19.7). Elastography has also been used to evaluate cirrhosis and transplant rejection.27 Furthermore, ultrasound elastography can be used to monitor the effects of high intensity focused ultrasound during treatment. Treated lesions become stiffer, which manifests as decreased strain on elastograms.29
Orbital ultrasound elastography also appears to be feasible. For example, a study in blind patients showed that the anterior vitreous has intermediate elasticity, while the posterior vitreous has low elasticity. The elasticity of the rectus muscles varies with position, being higher in neutral position than in adduction or abduction.30
Fusion imaging
Fusion imaging is a process in which previously obtained corresponding MRI, CT, or positron emission tomography/CT images are overlaid upon or displayed adjacent to an ultrasound image during real-time scanning. This method is based on global positioning system technology and requires sensors situated next to the patient for coregistration. This technology allows ultrasound-guided procedures to be carried out for lesions that are otherwise inconspicuous on ultrasound. This modality can depict the position of various anatomical structures with respect to the surgical approach. Fusion of intraoperative ultrasound with preoperative CT or MRI has proven useful for guiding resections of complex frontobasal tumors affecting the orbits.31
Remote and robotic ultrasound
There is a trend for compact, portable ultrasound units that can produce images of diagnostic quality for immediate expert interpretation over long distances. Lightweight six degrees-of-freedom robots have been developed for such application.32 The robot holds and scans an ultrasound probe on a distant patient according to the expert hand movement. The acquired images can be transmitted via satellite or integrated digital network lines. These systems are feasible and effective, but are currently under testing.
High-intensity focused ultrasound
HIFU has been used to treat glaucoma, intraocular tumors, retinal detachment, and failed trabeculectomies.8,33–35 Miniature circular transducers have been developed for the purpose of inducing coagulative necrosis of the ciliary body for treating glaucoma. Success in reducing intraocular pressure by using HIFU has been achieved in over 80% and 50% of patients at 3 months and 12 months post-treatment, respectively.34 Similarly, postoperative non functional trabeculectomy filtering blebs have been successfully treated with HIFU.35
The most common complications of ocular HIFU procedures include an immediate rise in intraocular pressure and mild iritis. The development of cataracts, phthisis bulbi, and thinning of the sclera are less common complications, with incidences of about 2.5% or less.35
Photoacoustic imaging
Photoacoustic (PA) imaging is a non-invasive modality that combines optical and ultrasound imaging technology. The photoacoustic effect is a phenomenon in which ultrasound waves are generated by light absorbers in soft tissues exposed to a very short duration (~nanoseconds) pulsed laser beam in the near-infrared (NIR) region (wavelengths 700 to 1000 nm).36 These photoacoustic (PA) waves can be detected by one or more ultrasound sensors located on the surface of the structure being imaged. PA imaging has potential applications in early detection of cancer (Figure 19.8), guiding biopsy, imaging with targeted nanoparticles, and blood oxygen level determination.36 In addition, the differential tissue absorption spectra in the NIR can enable PA spectroscopy and functional imaging.37
1 Zeiss E. Ueber Linsenveraenderungen an herausgenommenen Rinderlinsen durch Ultraschalleinwirkung. Graefes Arch Ophthalmol. 1938;139:301-322.
2 Thijssen JM. The history of ultrasound techniques in ophthalmology. Ultrasound Med Biol. 1993;19(8):599-618.
3 Mundt GHJr, Hughes WFJr. Ultrasonics in ocular diagnosis. Am J Ophthalmol. 1956;41(3):488-498.
4 Ossoinig K. [On the ultrasonic diagnosis of eye tumors. (Clinical and experimental studies with saw-tooth recordings)]. Klin Monbl Augenheilkd. 1965;146:321-337.
5 Pavlin CJ, Harasiewicz K, Sherar MD, et al. Clinical use of ultrasound biomicroscopy. Ophthalmology. 1991;98(3):287-295.
6 Coleman DJ, Silverman RH, Chabi A, et al. High-resolution ultrasonic imaging of the posterior segment. Ophthalmology. 2004;111(7):1344-1351.
7 McNicholas MM, Brophy DP, Power WJ, et al. Ocular sonography. AJR Am J Roentgenol. 1994;163(4):921-926.
8 Aptel F, Charrel T, Palazzi X, et al. Histologic effects of a new device for high-intensity focused ultrasound cyclocoagulation. Invest Ophthalmol Vis Sci. 2010;51(10):5092-5098.
9 Eccardt PC, Niederer K. Micromachined ultrasound transducers with improved coupling factors from a CMOS compatible process. Ultrasonics. 2000;38(1–8):774-780.
10 Foster FS, Pavlin CJ, Harasiewicz KA, et al. Advances in ultrasound biomicroscopy. Ultrasound Med Biol. 2000;26(1):1-27.
11 Lieb WE. Color Doppler imaging of the eye and orbit. Radiol Clin North Am. 1998;36(6):1059-1071.
12 Tranquart F, Berges O, Koskas P, et al. Color Doppler imaging of orbital vessels: personal experience and literature review. J Clin Ultrasound. 2003;31(5):258-273.
13 Schlottmann K, Fuchs-Koelwel B, Demmler-Hackenberg M, et al. High-frequency contrast harmonic imaging of ophthalmic tumor perfusion. AJR Am J Roentgenol. 2005;184(2):574-578.
14 Bouakaz A, Frigstad S, Ten Cate FJ, et al. Super harmonic imaging: a new imaging technique for improved contrast detection. Ultrasound Med Biol. 2002;28(1):59-68.
15 van Neer PL, Matte G, Danilouchkine MG, et al. Super-harmonic imaging: development of an interleaved phased-array transducer. IEEE Trans Ultrason Ferroelectr Freq Control. 2010;57(2):455-468.
16 Bouakaz A, Cate F, de Jong N. A new ultrasonic transducer for improved contrast nonlinear imaging. Phys Med Biol. 2004;49(16):3515-3525.
17 Lemke AJ, Hosten N, Richter M, et al. Contrast-enhanced color Doppler sonography of uveal melanomas. J Clin Ultrasound. 2001;29(4):205-211.
18 Han SS, Chang SK, Yoon JH, et al. The use of contrast-enhanced color Doppler ultrasound in the differentiation of retinal detachment from vitreous membrane. Korean J Radiol. 2001;2(4):197-203.
19 Jespersen SK, Wilhjelm JE, Sillesen H. Multi-angle compound imaging. Ultrason Imaging. 1998;20(2):81-102.
20 Entrekin RR, Porter BA, Sillesen HH, et al. Real-time spatial compound imaging: application to breast, vascular, and musculoskeletal ultrasound. Semin Ultrasound CT MR. 2001;22(1):50-64.
21 Ramos GA, Ylagan MV, Romine LE, et al. Diagnostic evaluation of the fetal face using 3-dimensional ultrasound. Ultrasound Q. 2008;24(4):215-223.
22 Wong HS, Parker S, Tait J, et al. Antenatal diagnosis of anophthalmia by three-dimensional ultrasound: a novel application of the reverse face view. Ultrasound Obstet Gynecol. 2008;32(1):103-105.
23 Romero JM, Finger PT, Rosen RB, et al. Three-dimensional ultrasound for the measurement of choroidal melanomas. Arch Ophthalmol. 2001;119(9):1275-1282.
24 Finger PT, Garcia JPJr, Pro MJ, et al. “C-scan” ultrasound imaging of optic nerve extension of retinoblastoma. Br J Ophthalmol. 2005;89(9):1225-1226.
25 Garcia JPJr, Finger PT, Kurli M, et al. 3D ultrasound coronal C-scan imaging for optic nerve sheath meningioma. Br J Ophthalmol. 2005;89(2):244-245.
26 Garcia JPJr, Garcia PM, Rosen RB, et al. Optic nerve measurements by 3D ultrasound-based coronal “C-scan” imaging. Ophthalmic Surg Lasers Imaging. 2005;36(2):142-146.
27 Garra BS. Imaging and estimation of tissue elasticity by ultrasound. Ultrasound Q. 2007;23(4):255-268.
28 Ginat DT, Destounis SV, Barr RG, et al. US elastography of breast and prostate lesions. Radiographics. 2009;29(7):2007-2016.
29 Souchon R, Rouviere O, Gelet A, et al. Visualisation of HIFU lesions using elastography of the human prostate in vivo: preliminary results. Ultrasound Med Biol. 2003;29(7):1007-1015.
30 Detorakis ET, Drakonaki EE, Tsilimbaris MK, et al. Real-time ultrasound elastographic imaging of ocular and periocular tissues: a feasibility study. Ophthalmic Surg Lasers Imaging. 2010;41(1):135-141.
31 Lohnstein PU, Schipper J, Berlis A, et al. [Sonography aided computer assisted surgery (SACAS) in orbital surgery]. HNO. 2007;55(10):778-784.
32 Delgorge C, Courreges F, Al Bassit L, et al. A tele-operated mobile ultrasound scanner using a light-weight robot. IEEE Trans Inf Technol Biomed. 2005;9(1):50-58.
33 Silverman RH, Vogelsang B, Rondeau MJ, et al. Therapeutic ultrasound for the treatment of glaucoma. Am J Ophthalmol. 1991;111(3):327-337.
34 Valtot F, Kopel J, Haut J. Treatment of glaucoma with high intensity focused ultrasound. Int Ophthalmol. 1989;13(1–2):167-170.
35 Yablonski M, Masonson HN, el-Sayyad F, et al. Use of therapeutic ultrasound to restore failed trabeculectomies. Am J Ophthalmol. 1987;103(4):492-496.
36 Keerthi SV, Bhargava KC, Navalgund AR, et al. Basics and clinical applications of photoacoustic imaging. Ultrasound Clin. 2009;4(3):403-429.
37 Vogel A, Venugopalan V. Mechanisms of pulsed laser ablation of biological tissues. Chem Rev. 2003;103(2):577-644.