CHAPTER 5 Functional anatomy of the musculoskeletal system
The skeletal system consists of the specialized supporting connective tissues of the bony skeleton and the associated tissues of joints, including cartilage. Cartilage is the fetal precursor tissue in the development of many bones; it also supports non-skeletal structures, as in the ear, larynx and tracheobronchial tree. Bone provides a rigid framework which protects and supports most of the soft tissues of the body and acts as a system of struts and levers which, through the action of attached skeletal muscles, permits movement of the body. Bones of the skeleton are connected with each other at joints which, according to their structure, allow varying degrees of movement. Some joints are stabilized by fibrous tissue connections between the articulating surfaces, while others are stabilized by tough but flexible ligaments. Skeletal muscles are attached to bone by strong flexible, but inextensible, tendons which insert into bone tissue. The entire assembly forms the musculoskeletal system; all its cells are related members of the connective tissue family and are derived from mesenchymal stem cells.
CARTILAGE
MICROSTRUCTURE OF CARTILAGE
Extracellular matrix
The extracellular matrix is composed of collagen and, in some cases, elastic fibres, embedded in a highly hydrated but stiff ground substance (Fig. 5.1). The components are unique to cartilage, and endow it with unusual mechanical properties. The ground substance is a firm gel, rich in carbohydrates and predominantly acidic. The chemistry of the ground substance is complex. It consists mainly of water and dissolved salts, held in a meshwork of long interwoven proteoglycan molecules together with various other minor constituents, mainly proteins or glycoproteins.
Synthesis of matrix
Chondrocytes synthesize and secrete all of the major components of the matrix. Collagen is synthesized within the rough endoplasmic reticulum in the same way as in fibroblasts, except that type II rather than type I procollagen chains are made. These assemble into triple helices and some carbohydrate is added at this stage. After transport to the Golgi apparatus, where further glycosylation occurs, they are secreted as procollagen molecules into the extracellular space. Here, terminal registration peptides are cleaved from their ends, so forming tropocollagen molecules, and final assembly into collagen fibres takes place. Core proteins of the proteoglycan complexes are also synthesized in the rough endoplasmic reticulum and addition of GAG chains begins. The process is completed in the Golgi complex. Hyaluronan, which lacks a protein core, is synthesized by enzymes on the surface of the chondrocyte; it is not modified post-synthetically, and is extruded directly into the matrix without passing through the endoplasmic reticulum.
Hyaline cartilage
Hyaline cartilage has a homogeneous glassy, bluish opalescent appearance. It has a firm consistency and some elasticity. Costal, nasal, some laryngeal, tracheobronchial, all temporary (developmental) and most articular, cartilages are hyaline. The arytenoid cartilage changes from hyaline at its base, to elastic cartilage at its apex. Size, shape and arrangement of cells, fibres and proteoglycan composition vary at different sites and with age. The chondrocytes are flat near the perichondrium and rounded or angular, deeper in the tissue. They are often grouped in pairs, sometimes more, forming cell nests (isogenous cell groups) which are daughter cells of a common parent chondroblast: apposing cells have a straight outline. The matrix is typically basophilic (Fig. 5.2) and metachromatic, particularly in the lacunar capsule, where recently formed, territorial matrix borders the lacuna of a chondrocyte. The paler-staining interterritorial matrix between cell nests is older synthetically. Fine collagen fibres are arranged in a basket-like network (Fig. 5.3), but are often absent from a narrow zone immediately surrounding the lacuna. An isogenous cell group, together with the enclosing pericellular matrix, is sometimes referred to as a chondron.
Articular hyaline cartilage
Articular hyaline cartilage covers articular surfaces in synovial joints (Fig. 5.4). It provides an extremely smooth, resistant surface bathed by synovial fluid, which allows almost frictionless movement. Its elasticity, together with that of other articular structures, dissipates stresses, and gives the whole articulation some flexibility, particularly near extremes of movement. Articular cartilage is particularly effective as a shock-absorber, and resists the large compressive forces generated by weight transmission, especially during movement.
Articular cartilage does not ossify. It varies from 1 to 7 mm in thickness and is moulded to the shape of the underlying bone, indeed it often accentuates and modifies the surface geometry of the bone. It is thickest centrally on convex osseous surfaces, and the reverse is true of concave surfaces. Its thickness decreases from maturity to old age. The surface of articular cartilage lacks a perichondrium; synovial membrane overlaps and then merges into its structure circumferentially (see Fig. 5.32).
Adult articular cartilage shows a structural zonation with increasing depth from the surface. The arrangement of collagen fibres has been variously described as plexiform, helical, or in the form of serial arcades which radiate from the deepest zone to the surface, where they follow a short tangential course before returning radially. If the surface of an articular cartilage is pierced by a needle, a longitudinal split-line remains after withdrawal. For any given joint, the patterns of split-lines are constant and distinctive and follow the predominant directions of collagen bundles in tangential zones of cartilage. These patterns may reveal tension trajectories set up in surrounding cartilage during joint movement.
The sequence of structural features outlined above is also typical of cartilaginous growth plates (see p. 95). During radial epiphysial growth, the extension of endochondral ossification into overlying calcified cartilage starts with the development of isogenous groups followed by the appearance of hypertrophic cells arranged in vertical columns. This ceases in maturity, but the zones persist throughout life. The same terminal mechanism also occurs in bones which lack epiphyses.
Fibrocartilage
Fibrocartilage is dense, fasciculated, opaque white fibrous tissue. It contains fibroblasts and small interfascicular groups of chondrocytes. The cells are ovoid and surrounded by concentrically striated matrix (Fig. 5.5). When present in quantity, as in intervertebral discs, fibrocartilage has great tensile strength and appreciable elasticity. In lesser amounts, as in articular discs, the glenoid and acetabular labra, and the cartilaginous lining of bony grooves for tendons and some articular cartilages, it provides strength, elasticity and resistance to repeated pressure and friction. It is resistant to degenerative change.
The articular surfaces of bones which ossify in mesenchymal membranes (e.g. squamous temporal, mandible and clavicle) are covered by white fibrocartilage. The deep layers, adjacent to hypochondral bone, resemble calcified regions of the radial zone of hyaline articular cartilage. The superficial zone contains dense parallel bundles of thick collagen fibres, interspersed with typical dense connective tissue fibroblasts and little ground substance. Fibre bundles in adjacent layers alternate in direction, as they do in the cornea. A transitional zone of irregular bundles of coarse collagen and active fibroblasts separates the superficial and deep layers. The fibroblasts are probably involved in elaboration of proteoglycans and collagen, and may also constitute a germinal zone for deeper cartilage. Fibre diameters and types may differ at different sites according to the functional load.
Elastic cartilage
Elastic cartilage occurs in the external ear, corniculate cartilages, epiglottis and apices of the arytenoids. It contains typical chondrocytes, but its matrix is pervaded by yellow elastic fibres, except around lacunae (where it resembles typical hyaline matrix with fine type II collagen fibrils) (Fig. 5.6). Its elastic fibres are irregularly contoured and show no periodic banding. Most sites in which elastic cartilage occurs have vibrational functions, such as laryngeal sound wave production, or the collection and transmission of sound waves in the ear. Elastic cartilage is resistant to degeneration; it can regenerate to a limited degree following traumatic injury, e.g. the distorted repair of a ‘cauliflower ear’.
DEVELOPMENT AND GROWTH OF CARTILAGE
Cartilage is usually formed in embryonic mesenchyme. Mesenchymal cells proliferate and become tightly packed: the shape of their condensation foreshadows that of the future cartilage. They also become rounded, with prominent round or oval nuclei and a low cytoplasm: nucleus ratio. Adjacent cells are linked by gap junctions. Each cell next secretes a basophilic halo of matrix, composed of a delicate network of fine type II collagen filaments, type IX collagen and cartilage proteoglycan core protein, i.e. it differentiates into a chondroblast (Fig. 5.7). In some sites, continued secretion of matrix separates the cells, producing typical hyaline cartilage. Elsewhere, many cells become fibroblasts: collagen synthesis predominates and chondroblastic activity appears only in isolated groups or rows of cells which become surrounded by dense bundles of collagen fibres to form white fibrocartilage. In yet other sites, the matrix of early cellular cartilage is permeated first by anastomosing oxytalan fibres, and later by elastin fibres. In all cases, developing cartilage is surrounded by condensed mesenchyme which differentiates into a bilaminar perichondrium. The cells of the outer layer become fibroblasts and secrete a dense collagenous matrix lined externally by vascular mesenchyme. The cells of the inner layer contain differentiated, but mainly resting, chondroblasts or prechondroblasts.
BONE
MACROSCOPIC ANATOMY OF BONE
Macroscopically, living bone is white. Its texture is either dense like ivory (compact bone), or honeycombed by large cavities (trabecular, cancellous or spongy bone), where the bony element is reduced to a latticework of bars and plates (trabeculae) (Fig. 5.8, Fig. 5.9). Compact bone is usually limited to the cortices of mature bones (cortical bone) and is of great importance in providing their strength. Its thickness and architecture vary for different bones, according to their overall shape, position and functional roles. The cortex plus the hollow medullary canal of long bones allows combination of strength with low weight. Cancellous bone is usually internal, giving additional strength to cortices and supporting the bone marrow. Bone forms a reservoir of metabolic calcium (99% of body calcium is in the bony skeleton) and phosphate which is under hormonal and cytokine control.
Bones vary not only in their primary shape but also in lesser surface details, or secondary markings, which appear mainly in postnatal life. Most bones display features such as elevations and depressions (fossae), smooth areas and rough ridges. Numerous names are used to describe these secondary features. Some articular surfaces are called fossae (e.g. the glenoid fossa); lengthy depressions are grooves or sulci (e.g. the humeral bicipital sulcus); a notch is an incisura, and an actual gap is a hiatus. A large projection is termed a process or, if elongated and slender or pointed, a spine. A curved process is a hamulus or cornu (e.g. the pterygoid hamuli of the sphenoid bone and the cornua of the hyoid). A rounded projection is a tuberosity or tubercle, and occasionally a trochanter. Long elevations are crests, or lines, if they are less developed; crests are wider and present boundary edges or lips. An epicondyle is a projection close to a condyle and is usually a site where the common tendon of a superficial muscle group or the collateral ligament of the adjacent joint are attached. The terms protuberance, prominence, eminence and torus are less often applied to certain bony projections. The expanded proximal ends of many long bones are often termed the ‘head’ or caput (e.g. humerus, femur, radius). A hole in bone is a foramen, and becomes a canal when lengthy. Large holes may be called apertures or, if covered largely by connective tissue, fenestrae. Clefts in or between bones are fissures. A lamina is a thin plate; larger laminae may be called squamae (e.g. the temporal squama). Large areas on many bones are featureless and often smoother than articular surfaces, from which they differ because they are pierced by many visible vascular foramina. This texture occurs where muscle is directly attached to bone, and small blood vessels pass through the foramina from bone to muscle, and perhaps vice versa. Areas covered only by periosteum are similar, but vessels are less numerous.
MICROSTRUCTURE OF BONE
Bone matrix
Bone matrix is the mineralized extracellular material of bone; like general connective tissues, it consists of a ground substance in which numerous collagen fibres are embedded, usually ordered in parallel branching arrays (Fig. 5.10). In mature bone, the matrix is moderately hydrated, and 10–20% of its mass is water. Of its dry weight, 60–70% is made up of inorganic, mineral salts (mainly microcrystalline calcium and phosphate hydroxides, hydroxyapatite (see below), approximately 30% is collagen and the remainder is non-collagenous protein and carbohydrate, mainly conjugated as glycoproteins. The proportions of these components vary with age, location and metabolic status.
Osteoblasts
Osteoblasts are derived from osteoprogenitor (stem) cells of mesenchymal origin, which are present in the bone marrow and other connective tissues. They proliferate and differentiate, stimulated by bone morphogenetic proteins (BMPs), into osteoblasts prior to bone formation. Osteoblasts are basophilic, roughly cuboidal mononuclear cells 15–30 μm across. Ultrastructurally, they have features typical of protein-secreting cells. They are found on the forming surfaces of growing or remodelling bone, where they constitute a covering layer (Fig. 5.11). In relatively quiescent adult bones they appear to be present mostly on endosteal rather than periosteal surfaces, but they also occur deep within compact bone where osteons are being remodelled. They are responsible for the synthesis, deposition and mineralization of the bone matrix, which they secrete. Once embedded in the matrix, they become osteocytes.
Osteoblasts play a key role in the hormonal regulation of bone resorption, since they express receptors for parathyroid hormone (PTH), 1,25-dihydroxy vitamin D3 and other promoters of bone resorption. During bone resorption, osteoblasts promote osteoclast differentiation via PTH-activated expression of cell surface RANKL, which binds to RANK on immature osteoclasts, establishes cell–cell contact and triggers contact-dependent osteoclast differentiation. In the presence of PTH, osteoblasts also downregulate secretion of osteoprotegerin, a soluble decoy ligand with higher affinity for RANKL. In conditions favouring bone deposition, secreted osteoprotegerin blocks RANKL binding to RANK and restricts numbers of mature osteoclasts. (For a recent review, see Blair et al 2007.)
Osteocytes
Osteocytes constitute the major cell type of mature bone, and are scattered within its matrix, interconnected by numerous dendritic processes to form a complex cellular network (Fig. 5.12). They are derived from osteoblasts and are enclosed within their matrix but, unlike chondrocytes, they do not divide. Bone growth is appositional: new layers are added only to pre-existing surfaces and so, again unlike chondrocytes, osteocytes enclosed in lacunae do not secrete new matrix. The rigidity of mineralized bone matrix prevents internal expansion, which means that interstitial growth, which is characteristic of most tissues, does not occur in bone. Osteocytes retain contacts with each other and with cells at the surfaces of bone (osteoblasts and bone-lining cells) throughout their lifespan.
Mature, relatively inactive, osteocytes possess an ellipsoid cell body with the longest axis (25 μm) parallel to the surrounding bony lamella. The rather narrow rim of cytoplasm is faintly basophilic, contains relatively few organelles and surrounds an oval nucleus. Osteocytes in woven bone are larger and more irregular in shape (Fig. 5.13).
Osteoclasts
Functionally, osteoclasts are responsible for the local removal of bone during bone growth and subsequent remodelling of osteons and surface bone (see Fig. 5.25). They cause demineralization by proton release, which creates an acidic local environment, and organic matrix destruction by releasing lysosomal (cathepsin K) and non-lysosomal (e.g. collagenase) enzymes. Factors stimulating osteoclasts to resorb bone include osteoblast-derived signals; cytokines from other cells, e.g. macrophages and lymphocytes; blood-borne factors, e.g. parathyroid hormone and 1,25(OH)2 vitamin D3 (calcitriol). Calcitonin, produced by C cells of the thyroid follicle, reduces osteoclast activity.
Osteoclasts arise by fusion of monocytes derived from the bone marrow or other haemopoietic tissue. They probably share a common ancestor with macrophages within the granulocyte–macrophage lineage (see Fig. 4.12) but it is thought that they subsequently follow a distinct differentiation pathway.
Osteons
In woven, or bundle, bone, the collagen fibres and bone crystals are irregularly arranged. The diameters of the fibres vary, so that fine and coarse fibres intermingle, producing the appearance of the warp and weft of a woven fabric. Woven bone is typical of young fetal bones, but is also seen in adults during excessively rapid bone remodelling and repair of fractures (Fig. 5.14). It is formed by highly active osteoblasts during development, and is stimulated in the adult by fracture, growth factors, or prostaglandin E2.
Lamellar bone makes up almost all of an adult osseous skeleton (Fig. 5.15, Fig. 5.16). The precise arrangement of lamellae varies from site to site, particularly between compact cortical bone and the trabecular bone within. In many bones a few lamellae form continuous circumferential layers at the outer (periosteal) and inner (endosteal) surfaces. However, by far the greatest proportion of lamellae are arranged in concentric cylinders around neurovascular channels called Haversian canals, to form the basic units of bone tissue which are the Haversian systems or osteons. Osteons usually lie parallel with each other (Fig. 5.17) and, in elongated bones such as those of the appendicular skeleton, with the long axis of the bone. They may also spiral, branch or intercommunicate, and some end blindly.
The central Haversian canals of osteons vary in size, with a mean diameter of 50 μm; those near the marrow cavity are somewhat larger. Each canal contains one or two capillaries lined by fenestrated endothelium and surrounded by a basal lamina which also encloses typical pericytes. They usually contain a few unmyelinated and occasional myelinated axons. The bony surfaces of osteonic canals are perforated by the openings of osteocyte canaliculi and are lined by collagen fibres.
Haversian canals communicate with each other and directly or indirectly with the marrow cavity via vascular (nutrient) channels called Volkmann’s canals, which run obliquely or at right angles to the long axes of the osteons (Fig. 5.17). The majority of these channels appear to branch and anastomose, but some join large vascular connections with vessels in the periosteum and the medullary cavity.
Trabecular bone
The organization of trabecular (cancellous, spongy) bone is basically lamellar, as shown most clearly under polarized light (Fig. 5.18). It takes the form of branching and anastomosing curved plates, tubes and bars of various widths and lengths which surround marrow cavities and are lined by endosteal tissue (Fig. 5.8, Fig. 5.9). Their thickness ranges from 50 to 400 μm. In general, bone lamellae are oriented parallel with the adjacent bone surface, and the arrangement of cells and matrix is similar to that found in circumferential and osteonic bone. Thick trabeculae and regions close to compact bone may contain small osteons, but blood vessels do not otherwise lie within the bony tissue, and osteocytes therefore rely on canalicular diffusion from adjacent medullary vessels. In young bone, calcified cartilage may occur in the cores of trabeculae, but this is generally replaced by bone during subsequent remodelling.
Remodelling
Remodelling of the interior of a bone depends upon the balance of resorption and deposition of bone, i.e. on the balanced activities of osteoclasts and osteoblasts. Osteoclasts first excavate a cylindrical tunnel by concerted action. A ‘cutting cone’ is formed by groups of osteoclasts moving at 50 μm/day, followed by osteoblasts which fill in the space so created. The osteoblasts deposit new osteoid matrix concentrically around a centrally ingrowing blood vessel, starting at the peripheral surface of the tunnel. This forms a ‘closing cone’ with 4000 osteoblasts/mm2. Deposition of successive, concentric lamellae follows, as cohorts of osteoblasts become embedded in the matrix they secrete, and are succeeded by new osteoblasts which line the free surface thus created, and secrete the next layer. In this way the walls of resorption cavities are lined with new lamellar matrix, and the vascular channels are progressively narrowed (Fig. 5.19). The pattern and extent of remodelling is dictated by the mechanical loads applied to the bone.
A hypermineralized basophilic cement line marks a site of reversal from resorption to deposition. Formation of osteons does not end with growth but continues variably throughout life. Remnants of circumferential lamellae of old osteons form interstitial lamellae between newer osteons (Fig. 5.15, Fig. 5.16A).
Periosteum, endosteum and bone marrow
The outer surface of bone is covered by a condensed, fibrocollagenous layer, the periosteum. The inner surface is lined by a thinner, more cellular, endosteum. Osteoprogenitor cells, osteoblasts, osteoclasts and other cells important in the turnover and homeostasis of bone tissue lie in these layers.
The periosteal layer is tethered to the underlying bone by extrinsic collagen fibres, Sharpey’s fibres, which penetrate deep into the outer cortical bone tissue. It is absent from articular surfaces, and from the points of insertion of tendons and ligaments (entheses) (see Fig. 5.46). The periosteum is highly active during fetal development, when it generates osteoblasts for the appositional growth of bone. These cells form a layer, two to three cells deep, between the fibrous periosteum and new woven bone matrix. Osteoprogenitor cells within the mature periosteum are indistinguishable morphologically from fibroblasts. Periosteum is important in the repair of fractures: where it is absent, e.g. within the joint capsule of the femoral neck, fractures are slow to heal.
NEUROVASCULAR SUPPLY OF BONE
Vascular supply and lymphatic drainage
The osseous circulation supplies bone tissue, marrow, perichondrium, epiphysial cartilages in young bones, and, in part, articular cartilages. The vascular supply of a long bone depends on several points of inflow which feed complex and regionally variable sinusoidal networks within the bone. The sinusoids drain to venous channels which leave through all surfaces that are not covered by articular cartilage. The flow of blood through cortical bone in the shafts of long bones is mainly centrifugal (Fig. 5.20).
Medullary arteries in the shaft give off centripetal branches which feed a hexagonal mesh of medullary sinusoids that drain into a wide, thin-walled central venous sinus. They also possess cortical branches which pass through endosteal canals to feed fenestrated capillaries in osteons (Haversian systems). The central sinus drains into veins which retrace the paths of nutrient arteries, sometimes piercing the shaft elsewhere as independent emissary veins. Cortical capillaries follow the pattern of Haversian canals, and are mainly longitudinal with oblique connections via Volkmann’s canals (Fig. 5.17). At bone surfaces, cortical capillaries make capillary and venous connections with periosteal plexuses (Fig. 5.20). The latter are formed by arteries from neighbouring muscles which contribute vascular arcades with longitudinal links to the fibrous periosteum. From this external plexus a capillary network permeates the deeper, osteogenic periosteum. At muscular attachments, periosteal and muscular plexuses are confluent and the cortical capillaries then drain into interfascicular venules.
DEVELOPMENT AND GROWTH OF BONE
Intramembranous ossification
Intramembranous ossification is the direct formation of bone (membrane bone) within highly vascular sheets or ‘membranes’ of condensed primitive mesenchyme (Fig. 5.21). At centres of ossification, mesenchymal stem cells differentiate into osteoprogenitor cells which proliferate around the branches of a capillary network, forming incomplete layers of osteoblasts in contact with the primitive bone matrix. The cells are polarized because they secrete a fine mesh of collagen fibres and ground substance, osteoid, from the surface which faces away from the blood vessels. The earliest crystals appear in association with extracellular matrix vesicles produced by the osteoblasts; crystal formation subsequently extends into collagen fibrils in the surrounding matrix, producing an early labyrinth of woven bone, the primary spongiosa. As layers of calcifying matrix are added to these early trabeculae, the osteoblasts enclosed by matrix come to lie within primitive lacunae. New osteocytes retain intercellular contact by means of their fine cytoplasmic processes (dendrites) and, as these elongate, matrix condenses around them to form canaliculi.
As matrix secretion, calcification and enclosure of osteoblasts proceed, the trabeculae thicken and the intervening vascular spaces become narrower. Where bone remains trabecular, the process slows and the spaces between trabeculae become occupied by haemopoietic tissue. Where compact bone is forming, trabeculae continue to thicken and vascular spaces continue to narrow. Meanwhile the collagen fibres of the matrix, secreted on the walls of the narrowing spaces between trabeculae, become organized as parallel, longitudinal or spiral bundles, and the cells they enclose occupy concentric sequential rows. These irregular, interconnected masses of compact bone each have a central canal, and are called primary osteons (primary Haversian systems). They are later eroded, together with the intervening woven bone, and replaced by generations of mature (secondary) osteons.
Endochondral ossification
The first appearance of a centre of primary ossification (Fig. 5.22) occurs when chondroblasts deep in the centre of the primitive shaft enlarge greatly, and their cytoplasm becomes vacuolated and accumulates glycogen. Their intervening matrix is compressed into thin, often perforated, septa. The cells degenerate and may die, leaving enlarged and sometimes confluent lacunae (primary areolae) whose thin walls become calcified during the final stages (Fig. 5.23). Type X collagen is produced in the hypertrophic zone of cartilage. Matrix vesicles originating from chondrocytes in the proliferation zone are most evident in the intercolumnar regions, where they appear to initiate crystal formation. At the same time, cells in the deep layer of perichondrium around the centre of the cartilage model differentiate into osteoblasts and form a peripheral layer of bone. Initially, this periosteal collar, formed by intramembranous ossification within the perichondrium, is a thin-walled tube which encloses and supports the central shaft (Fig. 5.22, Fig. 5.23). As it increases in diameter it also extends towards both ends of the shaft.
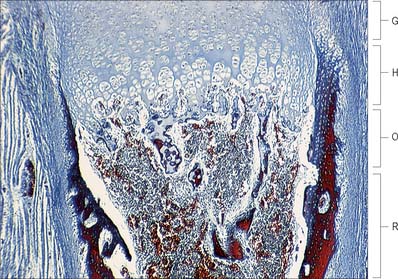
Fig. 5.23 The sequence of cellular events in endochondral ossification. This low magnification micrograph shows the primary ossification centre in a human fetal bone. See Fig. 5.26 for further details. G, growth zone; H, hypertrophic zone; O, ossification zone; R, remodelling zone.
The periosteal collar which overlies the calcified cartilaginous walls of degenerate chondrocyte lacunae is invaded from the deep layers of the periosteum (formerly perichondrium) by osteogenic buds. These are blind-ended capillary sprouts and are accompanied by osteoprogenitor cells and osteoclasts. The latter excavate newly formed bone to reach adjacent calcified cartilage where they continue to erode the walls of primary chondrocyte lacunae (Fig. 5.24, Fig. 5.25). This process leads to their fusion into larger and irregular communicating spaces, secondary areolae, which fill with embryonic medullary tissue (vascular mesenchyme, osteoblasts and osteoclasts, haemopoietic and marrow stromal cells, etc.). Osteoblasts attach themselves to the delicate residual walls of calcified cartilage and lay down osteoid which rapidly becomes confluent, forming a continuous lining of bone. Further layers of bone are added, enclosing young osteocytes in lacunae, and narrowing the perivascular spaces. Bone deposition on the more central calcified cartilage ceases as the formation of subperiosteal bone continues.
Osteoclastic erosion of the early bone spicules then creates a primitive medullary cavity in which only a few trabeculae, composed of bone with central cores of calcified cartilage (Fig. 5.24), remain to support the developing marrow tissues. These trabeculae soon become remodelled and replaced by more mature bone or by marrow. Meanwhile new, adjacent, cartilaginous regions undergo similar changes. Since these are most advanced centrally, and the epiphyses remain cartilaginous, the intermediate zones exhibit a temporospatial sequence of changes when viewed in longitudinal section (Fig. 5.26F). This region of dynamic change from cartilage to bone persists until longitudinal growth of the bone ceases.
Expansion of the cartilaginous extremity (usually an epiphysis) keeps pace with the growth of the rest of the bone both by appositional and interstitial growth. The growth zone expands in all dimensions. Growth in thickness of a developing long bone is caused by occasional transverse mitoses in its chondrocytes, and by appositional growth as a result of matrix deposition by cells from the perichondrial collar or ring at this level. The future growth plate therefore expands in concert with the shaft and adjacent future epiphysis. A zone of relatively quiescent chondrocytes (the resting zone) lies on the side of the plate closest to the epiphysis. An actively mitotic zone of cells faces towards the shaft of the bone: the more frequent divisions in the long axis of the bone soon create numerous longitudinal columns (palisades) of disc-shaped chondrocytes, each in a flattened lacuna (Fig. 5.26). Proliferation and column formation occurs in this zone of cartilage growth (the proliferative zone), and its continued longitudinal interstitial expansion provides the basic mode of elongation of a bone.
The columns of cells show increasing maturity towards the centre of the shaft, as their chondrocytes increase in size and accumulate glycogen. In the hypertrophic zone, energy metabolism is depressed at the level of the mineralizing front (Fig. 5.24). The lacunae are now separated by transverse and longitudinal walls, and the latter are impregnated with apatite crystals (the zone of calcified cartilage). The calcified partitions enter the zone of bone formation and are invaded by vascular mesenchyme containing osteoblasts, osteoclasts, etc. The partitions, especially the transverse ones, are then partly eroded while osteoid deposition, bone formation and osteocyte enclosure occur on the surfaces of the longitudinal walls. Lysis of calcified partitions is mediated by osteoclast (chondroclast) action, aided by cells associated with the terminal buds of vascular sinusoids which occupy, and come into close contact with, each incomplete columnar trabecular framework.
Postnatal growth and maintenance
Lamellar bone forms at variable rates which reflect the slow turnover of osteons throughout adult life. A resorption canal (cutting cone) is typically 2 mm long and takes 1–3 months to form; a new osteon (closing cone) forms in a similar period (Fig. 5.19). Internal remodelling continuously supplies young osteons with labile calcium reserves, and provides a malleable bony architecture that is responsive to changing patterns of stress. The remodelling unit in cancellous bone, equivalent to the secondary osteon of compact bone, is the bone structural unit: it has an average thickness of 40–70 μm and an average length of 100 μm, but may be more extensive and irregular in shape.
Vitamin C is essential for the adequate synthesis of collagen and matrix proteoglycans in connective tissues. When vitamin C is deficient, growth plates become thin, ossification almost stops, and metaphysial trabeculae and cortical bone are reduced in thickness. This causes fragility and delayed healing of fractures. Vitamin A is necessary for normal growth, and for a correct balance of deposition and removal of bone. Deficiency retards growth as a result of the failure of internal erosion and remodelling, particularly in the cranial base. Foramina are narrowed, sometimes causing pressure atrophy of contained nerves, and the cranial cavity and spinal canal may fail to expand with the central nervous system, which impairs nervous function. Conversely, excess vitamin A stimulates vascular erosion of growth cartilages, which become thin or totally lost, and longitudinal growth ceases. Retinoic acid, a vitamin A derivative, is involved in pattern formation in limb buds (see Ch. 51), and in the differentiation of osteoblasts.
Balanced endocrine functions are also essential to normal bone maturation, and disturbances in this balance may have profound effects. In addition to its role in calcium metabolism, excess parathyroid hormone (primary hyperparathyroidism) stimulates unchecked osteoclastic erosion of bone, particularly subperiosteally and later endosteally (osteitis fibrosa cystica). Growth hormone is required for normal interstitial proliferation in growth cartilages, and hence increase in stature. Termination of normal growth is imperfectly understood, but may involve a fall in hormone production or in the sensitivity of chondroblasts to insulin-like growth factors regulated by GH. Reduction of GH production in the young leads to quiescence and thinning of growth plates and hence pituitary dwarfism. Conversely, continued hypersecretion in the immature leads to gigantism, and in the adult results in thickening of bones by subperiosteal deposition; the mandible, hands and feet are the most affected, a condition known as acromegaly.
Growth of individual bones
Some bones, including carpal, tarsal, lacrimal, nasal, and zygomatic bones, inferior nasal conchae and auditory ossicles, ossify from a single centre which may appear between the eighth intrauterine week and the tenth year, a wide sequence for studying growth or estimating age. Most bones ossify from several centres, one of which appears in the centre of the future bone in late embryonic or early fetal life (seventh week to fourth month). Ossification progresses from the centres towards the ends, which are still cartilaginous at birth (Fig. 5.27). These terminal regions ossify from separate centres, which are sometimes multiple, and which appear between birth and the late teens: they are therefore secondary to the earlier primary centre from which much of the bone ossifies. This is the pattern in long bones, as well as in some shorter bones such as the metacarpals and metatarsals, and in the ribs and clavicles.
Variation in skeletal development occurs between individuals, sexes and possibly also races. The timing rather than the sequence of events varies, and females antedate males in all groups studied: differences which are perhaps insignificant before birth may be as great as two years in adolescence.
JOINTS
FIBROUS JOINTS
Fibrous joints lack intervening cartilage between the two bones, the articulation is therefore fixed and movement is very restricted. The three most definable types of fibrous articulation are sutures, gomphoses and syndesmoses (Fig. 5.28).
Suture
Sutures are restricted to the skull (see Ch. 26 for descriptions of individual sutures). In a suture, the two bones are separated by a layer of membrane-derived connective tissue. The sutural aspect of each bone is covered by a layer of osteogenic cells (cambial layer) overlaid by a capsular lamella of fibrous tissue which is continuous with the periosteum on both the endo- and ectocranial surfaces. The area between the capsular coverings contains loose fibrous connective tissue and decreases with age so that the osteogenic surfaces become apposed. On completion of growth, many sutures synostose and are obliterated. Synostosis occurs normally as the skull ages: it can begin in the early twenties and continues into advanced age. A schindylesis is a specialized suture in which a ridged bone fits into a groove on a neighboring element, e.g. where the cleft between the alae of the vomer receives the rostrum of the sphenoid.
CARTILAGINOUS JOINTS
Cartilaginous joints may be classified as primary (synchondrosis) or secondary (symphysis), depending upon the nature of the intervening cartilage. While the distinction between fibrous and cartilaginous joints is usually clear, some degree of admixture can occur in which either a predominantly fibrous articulation contains occasional islands of cartilage or a predominantly cartilaginous articulation contains aligned dense bundles of collagen. These joints tend to be less rigid than the fibrous articulations and some permit movement, albeit restricted in range (Fig. 5.29, Fig. 5.30).
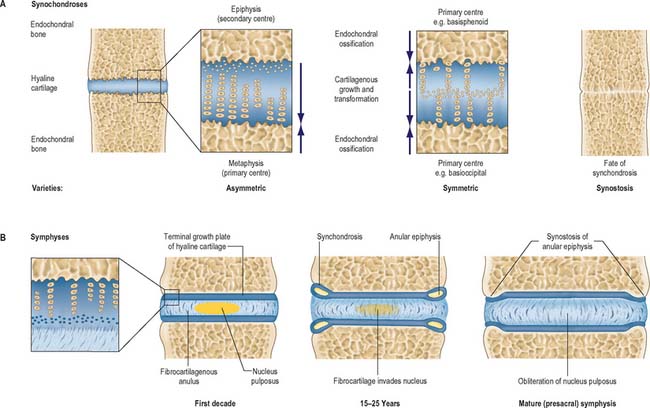
Fig. 5.29 Examples of varieties of cartilaginous joints (see also Fig. 5.30). A, Sectional view of the principal tissues involved, more detailed architecture and main growth patterns of symmetrical and asymmetrical synchondroses. Lesser degrees of asymmetry occur in some locations. Synostosis is the normal fate of almost all synchondroses when endochondral growth has ceased. B, Intervertebral symphyses (presacral), shown in section, displaying age-related changes. Partial or complete synostosis is the normal fate of sacral and coccygeal symphyses.
Primary cartilaginous joints
Primary cartilaginous joints or synchondroses occur where advancing centres of ossification are separated by an area of hyaline (non-articular) cartilage. They are present in all postcranial bones that form from more than one centre of ossification. Since hyaline cartilage retains the capability to ossify with age, synchondroses tend to synostose when growth is complete. Primary cartilaginous joints are almost exclusively associated with growth plates (see above): the detailed anatomy of specific joints is described in the appropriate topographical chapter. The location and growth of the spheno-occipital synchondrosis is discussed in Chapter 26.
Secondary cartilaginous joints
Secondary cartilaginous joints or symphyses are largely defined by the presence of an intervening pad or disc of fibrocartilage interposed between the articular hyaline cartilage that covers the ends of two bones. The pad or disc varies from a few millimetres to over a centimetre in width, and the whole region is generally bound by strong, tightly adherent, dense connective tissues. Collagenous ligaments extend from the periostea of the articulating bones across the symphysis and blend with the hyaline and fibrocartilaginous perichondria: they do not form a complete capsule and contain plexuses of afferent nerve terminals which also penetrate the periphery of the fibrocartilage. The combined strength of the ligaments and of the hyaline and fibrocartilage exceeds that of the associated bones, and is designed to withstand a range of stresses (compression, tension, shear and torsion). The range of movement in a symphysis is limited by the physical nature of the articulation and also by the restrictions imposed by other bones associated with the complex. Tears are usually the result of sudden, massive stresses that occur when the body is in an inappropriate posture.
SYNOVIAL JOINTS
Synovial articulations are quite readily differentiated from both fibrous and cartilaginous joints (Fig. 5.31). Although the bones involved are linked by a fibrous capsule (which usually has intrinsic ligamentous thickenings), and often by internal or external accessory ligaments, the articulating bony surfaces are generally not in direct continuity. They are covered by hyaline articular cartilage of varying thickness and precise topology; contact is strictly limited between these cartilaginous surfaces, which have a very low coefficient of friction, facilitating free movement. Smooth movement of the opposing articular surfaces is aided by a viscous synovial fluid, which acts as a lubricant, but is also concerned with cellular maintenance in the articular cartilages. The production of synovial fluid requires the presence of a synovial membrane which is one of the defining characteristics of the joint type.
Articular surfaces
Articular cartilages are moulded to bone (see Fig. 5.4), and variations in thickness often accentuate subjacent osseous surface shape. Typically, convex surfaces are thickest centrally, thinning peripherally, and concave surfaces are the reverse. The precise configuration, degree of congruence in various positions, and the dispositions of the surrounding capsule and ligaments, are all related to the types and ranges of movement permitted at a joint. Articular cartilage has no penetrating nerves or blood vessels (except occasional vascular loops). Its nutrition and maintenance therefore largely depend on diffusion from a peripheral vascular plexus in the synovial membrane (circulus vasculosus articuli), blood vessels in adjacent marrow spaces and synovial fluid: the relative importance of these contributions is uncertain.
The zone of articular cartilage next to the joint cavity is mainly a layer of collagen fibres arranged in various planes with small, oval chondrocytes lying deep to it in the matrix. Transmission electron microscopy of heavy metal stained preparations shows an interrupted electron-dense surface coat of a particulate or filamentous material, generally 0.03–0.1 μm thick, covering the cartilage. Synovial fluid and membranous debris, the product of chondrocytic necrosis, may contribute to this surface coat, which is transient in nature; the stable, permanent, articular surface is bounded by the most superficial collagen fibres. The ‘lamina splendens’, a structure that appears as a bright line at the free surface of articular cartilage when oblique sections are examined by negative phase contrast microscopy, is an artefact arising at the border between regions of different refractive index: it is not an anatomically distinct surface layer. The deeper zones of articular cartilage contain a highly complex, three-dimensional reticulum of interconnected fibrils, which have obvious functional implications.
Synovial membrane
Synovial membrane lines fibrous capsules and covers exposed osseous surfaces, intracapsular ligaments, bursae and tendon sheaths (Fig. 5.32). It does not cover intra-articular discs or menisci and stops at the margins of articular cartilages in a transitional zone that occupies the peripheral few millimetres of the cartilage. Synovial membrane secretes and absorbs a fluid that lubricates the movement between the articulating surfaces.
Synovial fluid
The composition of synovial fluid is consistent with it being mainly a dialysate of blood plasma. It contains protein (approximately 0.9 mg/100 ml) derived from the blood. It also contains hyaluronan, which is thought to be a significant determinant of the viscoelastic and thixotropic (flow rate dependent) properties of synovial fluid. A small proportion (approximately 2%) of synovial protein differs from plasma protein and is probably produced by synovial type B cells, and a very small proportion (approximately 0.5%) of synovial protein appears to be a specialized lubricating glycoprotein. Synovial fluid contains a few cells (approximately 60 per ml in resting human joints), including monocytes, lymphocytes, macrophages, synovial intimal cells and polymorphonuclear leukocytes: higher counts are found in young individuals. The amorphous metachromatic particles and fragments of cells and fibrous tissue found in synovial fluid are presumed to be produced by wear and tear.
Intra-articular menisci, discs and fat pads
The function of intra-articular fibrocartilages is uncertain. Deductions have been made from structural or phylogenetic data, aided by mechanical analogies; suggestions include shock absorption, improvement of fit between surfaces, facilitation of combined movements, checking of translation at joints such as the knee, deployment of weight over larger surface areas, protection of articular margins, facilitation of rolling movements, and spread of lubricant. The temporomandibular disc has attracted particular attention because of its exceptional, perhaps unique, design and biomechanical properties (see Ch. 31).
Classification
Synovial joints may be classified (Fig. 5.33) according to their shape. While this has some practical value, it should be remembered that they are merely variations, sometimes extreme, of two basic forms. Articular surfaces are never truly flat, or complete spheres, cylinders, cones or ellipsoids. They are better described as parts of a single ovoid surface or a complex construction of more than one such surface.
Factors influencing movement
Complexity of form
Most synovial joints have two surfaces and are simple articulations, e.g. the glenohumeral and interphalangeal joints. In some, one surface is wholly convex and greater in area than its opposing concave surface, and occasionally, both surfaces are concavoconvex. A joint with more than two articular surfaces is called a compound joint, e.g. the knee and the elbow. In all compound joints, articulating territories remain distinct. A synovial joint that contains an intra-articular disc or meniscus is called a complex joint, e.g. the tibiofemoral joint of the knee, the sternoclavicular and temporomandibular joints (see Fig. 5.31).
Degrees of freedom
Joint motion can be described by rotation and translation about three orthogonal axes. There are three possible rotations (axial, abduction–adduction, flexion–extension) and three possible translations (proximo-distal, mediolateral, anteroposterior). Each is a degree of freedom. For most joints, translations are negligible and do not need consideration (Fig. 5.34). A few joints have minor pure translatory movements, but most joint motion is by rotation.
For a uniaxial hinge joint with a single degree of freedom, a single unchanging axis of rotation would be predicted. However, because the shapes of joint surfaces are complex, there is a variable radius of curvature (Fig. 5.35) and consequently the axis of rotation will vary as joint movement progresses. When the variation is minor, e.g. the elbow, it is often appropriate to describe a mean position for the axis. In others, e.g. the knee, the situation is more complex.
Articular movements and mechanisms
Angulation
Axial rotation
Axial rotation is a widely, but often imprecisely, used term. Its restricted sense denotes movement around some notional ‘longitudinal’ axis which may even be in a separate bone, e.g. the dens of the second cervical vertebra, on which the atlas rotates. An axis may be approximately the centre of the shaft of a long bone, e.g. in medial and lateral humeral rotation (Fig. 5.34). It may be at an angle to the topographical axis of a bone, e.g. in movement of the radius on the ulna in pronation and supination, where the axis joins the centre of the radial head to the base of the ulnar styloid process, or in medial and lateral femoral rotation, where the axis joins the centre of the femoral head to a (disputed) point in the distal femur. In these examples, rotations can be independent adjunct motions, constituting a degree of freedom, or conjunct (coupled) rotations, which always accompany some other main movement as a consequence of articular geometry. Obligatory conjunct (coupled) motion is frequently combined with a degree of voluntary adjunct motion, the latter dictating what proportion of the motion occurs above the minimum obligatory component.
MUSCLE
Most cells possess cytoskeletal elements that are capable of lengthening or shortening and so enable the cell to change its shape. This capacity is important in a variety of cellular functions, e.g. locomotion, phagocytosis, mitosis and extension of processes. Proteins referred to as molecular motors (see Ch. 1) can effect changes of length much more rapidly than systems that are dependent on polymerization–depolymerization mechanisms (actin, tubulin), by using energy from the hydrolysis of adenosine 5′-triphosphate (ATP). Of these ATP-dependent systems, one of the most widespread is based on the interaction of actin and myosin.
CLASSIFICATION OF MUSCLE
Other contractile cells, including myofibroblasts and myoepithelial cells, are different in character and developmental origin. They contain smooth muscle-like contractile proteins and are found singly or in small groups.
SKELETAL MUSCLE
Shape and fibre architecture
It is possible to classify muscles based on their general shape and the predominant orientation of their fibres relative to the direction of pull (Fig. 5.36). Muscles with fibres that are largely parallel to the line of pull vary in form from flat, short and quadrilateral (e.g. thyrohyoid) to long and strap-like (e.g. sternohyoid, sartorius). In such muscles, individual fibres may run for the entire length of the muscle, or over shorter segments when there are transverse, tendinous intersections at intervals (e.g. rectus abdominis). In a fusiform muscle, the fibres may be close to parallel in the ‘belly’, but converge to a tendon at one or both ends. Where fibres are oblique to the line of pull, muscles may be triangular (e.g. temporalis, adductor longus) or pennate (feather-like) in construction. The latter vary in complexity from unipennate (e.g. flexor pollicis longus) and bipennate (e.g. rectus femoris, dorsal interossei) to multipennate (e.g. deltoid). Fibres may pass obliquely between deep and superficial aponeuroses, in a type of ‘unipennate’ form (e.g. soleus), or muscle fibres may start from the walls of osteofascial compartments and converge obliquely on a central tendon in circumpennate fashion (e.g. tibialis anterior). Muscles may exhibit a spiral or twisted arrangement (e.g. sternocostal fibres of pectoralis major and latissimus dorsi, which undergo a 180° twist between their medial and lateral attachments), or may spiral around a bone (e.g. supinator, which winds obliquely around the proximal radial shaft), or may contain two or more planes of fibres arranged in differing directions, a type of spiral sometimes referred to as cruciate (sternocleidomastoid, masseter and adductor magnus are all partially spiral and cruciate). Many muscles display more than one of these major types of arrangement, and show regional variations which correspond to contrasting, and in some cases independent, actions.
Size | Depth | Action |
---|---|---|
Attachment | ||
---|---|---|
Microstructure of skeletal muscle
The cellular units of skeletal muscle are enormous multinucleate muscle fibres (Fig. 5.37, Fig. 5.38) which develop by fusion of individual myoblasts (see below). Individual muscle fibres are long, cylindrical structures that tend to be consistent in size within a given muscle, but in different muscles may range from 10 to 100 μm in diameter and from millimetres to many centimeters in length. The cytoplasm of each fibre, sarcoplasm, is surrounded by a plasma membrane that is often called the sarcolemma. The contractile machinery is concentrated into myofibrils, long narrow structures (1–2 μm in diameter) that extend the length of the fibre and form the bulk of the sarcoplasm. Numerous moderately euchromatic, oval nuclei usually occupy a thin transparent rim of sarcoplasm between the myofibrils and the sarcolemma, and are especially numerous in the region of the neuromuscular junction (see Fig. 3.37). A transverse section of a muscle fibre may only reveal one or two nuclei, but there may be several hundred along the length of an entire fibre. Myogenic satellite cells lie between the sarcolemma and the surrounding basal lamina (see below).
The myofibrils are too tightly packed to be visible by routine light microscopy (see below). Of greater significance are transverse striations, produced by the alignment across the fibre of repeating elements, the sarcomeres, within neighbouring myofibrils. These cross-striations are usually evident in conventionally stained histological sections, but may be demonstrated more effectively using special stains. They are even more striking under polarized light when they appear as a pattern of alternating dark and light bands. The darker, anisotropic or A-bands, are birefringent and rotate the plane of polarized light strongly. The lighter, isotropic or I-bands, rotate the plane of polarized light to a negligible degree. In transverse section, the profiles of the fibres are usually polygonal (Fig. 5.38; see Fig. 5.40). The sarcoplasm often has a stippled appearance, because the transversely sectioned myofibrils are resolved as dots. Their packing density varies. In some muscles, e.g. the extrinsic muscles of the larynx, the muscle fibres tend not to be tightly packed, whereas in others, e.g. the group of jaw closing muscles, the fibres are closely packed and have rounded profiles.
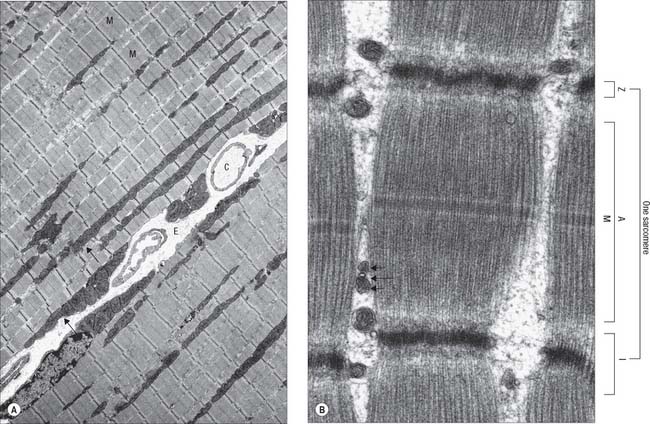
Fig. 5.40 The electron microscopic appearance of skeletal muscle in longitudinal section. A, Low-power view of parts of two adjacent muscle fibres, separated by endomysium (E) containing capillaries (C) and a peripherally-placed nucleus (N) in the fibre on the right. Mitochondria (arrows) are situated peripherally and between myofibrils (M). Myofibrils pack the cytoplasm, with their sarcomeres (contractile units) in register, as seen by the alignment of Z discs (dark transverse lines) across each muscle fibre. B, A sarcomere within a myofibril, and parts of two others. (A sarcomere is the distance between adjacent Z discs). Also seen are the A band, bisected by the M line, and I band, which here is almost obliterated in the contracted state (see Fig. 5.41). A triad is visible between myofibrils, comprised of a T-tubule (long arrow) and two terminal cisternae of sarcoplasmic reticulum (short arrows).
(Part A provided by courtesy of Professor Hans Hoppeler, Institute of Anatomy, University of Bern, Switzerland.)
In general, skeletal muscle fibres are large (there are a few exceptions, e.g. the intrinsic muscles of the larynx). This means that electron micrographs, unless of very low magnification, seldom show more than part of the interior of a fibre. Myofibrils, cylindrical structures about 1 μm diameter (Fig. 5.37), are the dominant ultrastructural feature of such micrographs. In longitudinal sections they appear as ribbons and are interrupted at regular intervals by thin, very densely stained transverse lines, which correspond to discsin the parent cylindrical structure. These are the Z-lines or, more properly, Z-discs (Zwischenscheiben = interval discs) that divide the myofibril into a linear series of repeating contractile units, sarcomeres, each of which is typically 2.2 μm long in resting muscle. At higher power, sarcomeres are seen to consist of two types of filament, thick and thin, organized into regular arrays (Fig. 5.38; Fig. 5.40). The thick filaments, which are approximately 15 nm in diameter, are composed mainly of myosin. The thin filaments, which are 8 nm in diameter, are composed mainly of actin. The arrays of thick and thin filaments form a partially overlapping structure in which electron density (as seen in the electron microscope) varies according to the amount of protein present. The A-band consists of the thick filaments, together with lengths of thin filaments that interdigitate with, and thus overlap, the thick filaments at either end (Fig. 5.40, Fig. 5.41). The central, paler region of the A-band, which is not penetrated by the thin filaments, is called the H-zone (Helle = light). At their centres, the thick filaments are linked together transversely by material that constitutes the M-line (Mittelscheibe = middle [of] disc), that is visible in most muscles. The I-band consists of the adjacent portions of two neighbouring sarcomeres in which the thin filaments are not overlapped by thick filaments. The thin filaments of adjacent sarcomeres are anchored in the Z-disc, which bisects the I-band. A third type of filament is composed of the elastic protein, titin.
The high degree of organization of the arrays of filaments is equally evident in electron micrographs of transverse sections (Fig. 5.41, Fig. 5.42). The thick myosin filaments form a hexagonal lattice. In the regions where they overlap the thin filaments, each myosin filament is surrounded by six actin filaments at the trigonal points of the lattice. In the I-band, the thin filament pattern changes from hexagonal to square as the filaments approach the Z-disc, where they are incorporated into a square lattice structure.
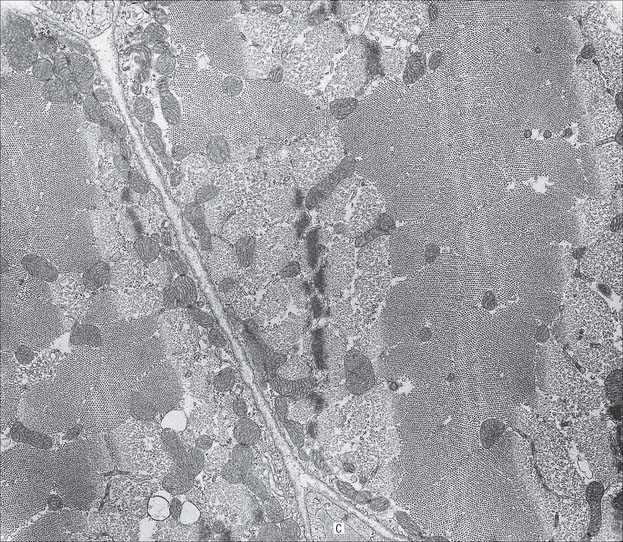
Fig. 5.42 Electron micrograph of skeletal muscle in transverse section, showing parts of two muscle fibres. Part of a capillary (C) is seen in transverse section in the endomysial space. The variation in the appearance of myofibrils in cross-section is explained in Fig. 5.41.
(Photograph by Professor Brenda Russell, Department of Physiology and Biophysics, University of Illinois at Chicago.)
Muscle proteins
Myosin, the protein of the thick filament, constitutes 60% of the total myofibrillar protein and is the most abundant contractile protein. The thick filaments of skeletal and cardiac muscle are 1.5 μm long. Their composition from myosin heavy and light chain assemblies is described in Chapter 1. The other components of myosin, the regulatory proteins tropomyosin and troponin, play a major part in the control of contraction. Actin is the next most abundant contractile protein and constitutes 20% of the total myofibrillar protein. In its filamentous form, F-actin, it is the principal protein of the thin filaments. A number of congenital myopathies result from gene mutations in components of the thin filament assembly (reviewed in Clarkson et al 2004). The third type of long sarcomeric filament connects the thick filaments to the Z-disc, and is formed by the giant protein, titin, which has a molecular mass in the millions. Single titin molecules span the half-sarcomere between the M-lines and the Z-discs, into which they are inserted. They have a tethered portion in the A-band, where they are attached to thick filaments as far as the M-line, and an elastic portion in the I-band. The elastic properties of titin endow the relaxed muscle fibre with passive resistance to stretching and with elastic recoil.
A number of proteins which are neither contractile nor regulatory are responsible for the structural integrity of the myofibrils, particularly their regular internal arrangement. A component of the Z-disc, α-actinin, is a rod-shaped molecule which anchors the plus-ends of actin filaments from adjacent sarcomeres to the Z-disc. Nebulin inserts into the Z-disc, associated with the thin filaments, and regulates the lengths of actin filaments. An intermediate filament protein characteristic of muscle, desmin, encircles the myofibrils at the Z-disc and, with the linking molecule plectrin, forms a meshwork that connects myofibrils together within the muscle fibre and to the sarcolemma. Myomesin holds myosin filaments in their regular lattice arrangement in the region of the M line. Dystrophin is confined to the periphery of the muscle fibre, close to the cytoplasmic face of the sarcolemma. It binds to actin intracellularly and is also associated with a large oligomeric complex of glycoproteins, the dystroglycan/sarcoglycan complex, that spans the membrane and links specifically with merosin, the α2 laminin isoform of the muscle basal lamina. This stabilizes the muscle fibre and transmits forces generated internally on contraction to the extracellular matrix.
Dystrophin is the product of the gene affected in Duchenne muscular dystrophy, a fatal disorder that develops when mutation of the gene leads to the absence of the protein. A milder form of the disease, Becker muscular dystrophy, is associated with a reduced size and/or abundance of dystrophin. Female carriers (heterozygous for the mutant gene) of Duchenne muscular dystrophy may also have mild symptoms of muscle weakness. At about 2500 kb, the gene is one of the largest yet discovered, which may account for the high mutation rate of Duchenne muscular dystrophy (approximately 35% of cases are new mutations). Other muscular dystrophies may involve deficiencies in proteins functionally associated with dystrophin, such as the dystroglycan/sarcoglycan complex or α2 laminin. The involvement in muscular dystrophy of defects in the dystrophin adhesion complex is reviewed in Batchelor & Winder (2006).
Other sarcoplasmic structures
Although myofibrils are the dominant ultrastructural feature of skeletal muscle, the fibres contain other organelles essential for cellular function, such as ribosomes, Golgi apparatus and mitochondria. Most of them are located around the nuclei, between myofibrils and the sarcolemma and, to a lesser extent, between the myofibrils. Mitochondria, lipid droplets and glycogen provide the metabolic support needed by active muscle. The mitochondria are elongated and their cristae are closely packed. The number of mitochondria in an adult muscle fibre is not fixed, but can increase or decrease quite readily in response to sustained changes in activity. Spherical lipid droplets, approximately 0.25 μm in diameter, are distributed uniformly throughout the sarcoplasm between myofibrils. They represent a rich source of energy that can be tapped only by oxidative metabolic pathways: they are therefore more common in fibres which have a high mitochondrial content and good capillary blood supply. Small clusters of glycogen granules are dispersed between myofibrils and among the thin filaments. In brief bursts of activity they provide an important source of anaerobic energy that is not dependent on blood flow to the muscle fibre.
Tubular invaginations of the sarcolemma penetrate between the myofibrils in a transverse plane at the limit of each A-band (Fig. 5.40). The lumina of these transverse (T-) tubules are thus in continuity with the extracellular space. At the ends of the muscle fibre, where force is transmitted to adjacent connective tissue structures, the sarcolemma is folded into numerous finger-like projections that strengthen the junctional region by increasing the area of attachment.
The sarcoplasmic reticulum (SR) is a specialized form of smooth endoplasmic reticulum and forms a plexus of anastomosing membrane cisternae that fills much of the space between myofibrils (Fig. 5.43). The cisternae expand into larger sacs, junctional sarcoplasmic reticulum or terminal cisternae, where they come into close contact with T-tubules, forming structures called triads (Fig. 5.40; Fig. 5.43). The membranes of the SR contain calcium–ATPase pumps that transport calcium ions into the terminal cisternae, where the ions are bound to calsequestrin, a protein with a high affinity for calcium, in dense storage granules. In this way, calcium can be accumulated and retained in the terminal cisternae at a much higher concentration than elsewhere in the sarcoplasm. Ca2+-release channels (ryanodine receptors) are concentrated mainly in the terminal cisternae and form one half of the junctional ‘feet’ or ‘pillars’ that bridge the SR and T-tubules at the triads. The other half of the junctional feet is the T-tubule receptor that constitutes the voltage sensor.
Vascular supply and lymphatic drainage
Mathes & Nahai (1981) have classified the gross vascular anatomy of muscles into five types according to the number and relative dominance of vascular pedicles which enter the muscle (Fig. 5.44). This classification has important surgical relevance in determining which muscles will survive and therefore be useful for pedicled or free tissue transfer procedures using techniques of plastic and reconstructive surgery. Type I muscles possess a single vascular pedicle supplying the muscle belly, e.g. tensor faciae latae (supplied by the ascending branch of the lateral circumflex femoral artery) and gastrocnemius (supplied by the sural artery). Type II muscles are served by a single dominant vascular pedicle and several minor pedicles, and can be supported on a minor pedicle as well as the dominant pedicle, e.g. gracilis (supplied by the medial circumflex femoral artery in the dominant pedicle). Type III muscles are supplied by two separate dominant pedicles each from different source arteries, e.g. rectus abdominus (supplied by the superior and inferior epigastric arteries) and gluteus maximus (supplied by the superior and inferior gluteal arteries). Type IV muscles have multiple small pedicles which, in isolation, are not capable of supporting the whole muscle, e.g. sartorius and tibialis anterior: about 30% survive reduction onto a single vascular pedicle. Type V muscles have one dominant vascular pedicle and multiple secondary segmental pedicles, e.g. latissimus dorsi (supplied by the thoracodorsal artery as the primary pedicle, and thoracolumbar perforators from the lower six intercostal arteries and the lumbar arteries as the segmental supply), and pectoralis major (supplied by the pectoral branch of the thoracoacromial axis as the dominant pedicle, and anterior perforators from the internal thoracic vessels as the segmental supply).
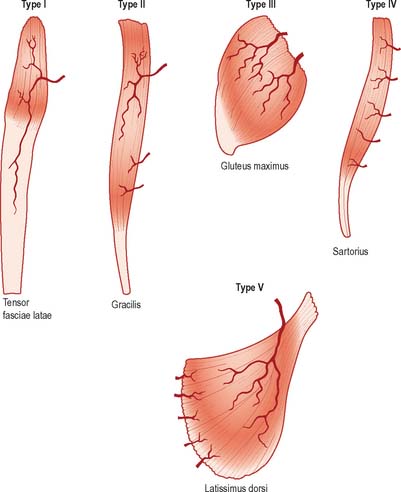
Fig. 5.44 Classification of muscles according to their blood supply.
(By permission from Cormack GC, Lamberty BGH 1994 The Arterial Anatomy of Skin Flaps, 2nd edn. Edinburgh: Churchill Livingstone.)
In muscle cross-sections, the number of capillary profiles found adjacent to fibres usually varies from 0 to 3. Fibres that are involved in sustained activities, such as posture, are served by a denser capillary network than fibres that are recruited only infrequently. It is common for muscles to receive their arterial supply via more than one route. The accessory arteries penetrate the muscle at places other than the hilum, and ramify in the same way as the principal artery, forming vascular territories. The boundaries of adjacent territories are spanned by anastomotic vessels, sometimes at constant calibre, but more commonly through reduced-calibre arteries or arterioles which are referred to as ‘choke vessels’ (see Ch. 6). These arterial arcades link the territories into a continuous network.
Because of the potential for relative movement within muscle groups, vessels tend not to cross between muscles, but radiate to them from more stable sites or cross at points of fusion. Where a muscle underlies the skin, vessels bridge between the two. These may be primarily cutaneous vessels, i.e. they supply the skin directly, but contribute small branches to the muscle as they pass through it, or they may be the terminal branches of intramuscular vessels which leave the muscle to supplement the cutaneous blood supply. The latter are less frequent where the muscle is mobile under the deep fascia. The correspondence between the vascular territories in the skin and underlying tissues gave rise to the concept of angiosomes, whichare composite blocks of tissue supplied by named distributing arteries and drained by their companion veins (see Ch. 6).
The pressure exerted on valved intramuscular veins during muscular contraction functions as a ‘muscle pump’ that promotes venous return to the heart. In some cases this role appears to be amplified by veins which pass through the muscle after originating elsewhere in superficial or deep tissues (see Ch. 79). The extent to which the muscle capillary bed is perfused can be varied in accordance with functional demand. Arteriovenous anastomoses, through which blood can be returned directly to the venous system without traversing the capillaries, provide an alternative, regulated pathway.
Innervation
Within muscles, nerves travel through the epimysial and perimysial septa before entering the fine endomysial tissue around the muscle fibres. α-Motor axons branch repeatedly before they lose their myelinated sheaths and terminate in a narrow zone towards the centre of the muscle belly known as the motor point. Clinically, this is the place on a muscle from which it is easiest to elicit a contraction with stimulating electrodes. Long muscles generally have two or more terminal, or end-plate bands, because many muscle fibres do not run the full length of an anatomical muscle. The terminal branch of an α-motor axon contacts a muscle fibre at a specialized synapse, the neuromuscular junction (see Fig. 3.37). It gives off several short, tortuous branches each ending in an elliptical area, the motor end plate. The underlying discoidal patch of sarcolemma, the sole plate or subneural apparatus, is thrown into deep synaptic folds. This discrete type of neuromuscular junction is an example of an en plaque ending and is found on muscle fibres which are capable of propagating action potentials. A different type of ending is found on slow tonic muscle fibres, which do not have this capability, e.g. in the extrinsic ocular muscles, where slow tonic fibres form a minor component of the anatomical muscle. In this case the propagation of excitation is taken over by the nerve terminals, which branch over an extended distance to form a number of small neuromuscular junctions (en grappe endings). Some muscle fibres of this type receive the terminal branches of more than one motor neurone. The terminals of the γ-efferents that innervate the intrafusal muscle fibres of the neuromuscular spindle also take a variety of different forms.
The terminal branches of α-motor axons are normally in a ‘one-to-one’ relationship with their muscle fibres: a muscle fibre receives only one branch, and any one branch innervates only one muscle fibre. When a motor neurone is excited, an action potential is propagated along the axon and all of its branches to all of the muscle fibres that it supplies. The motor neurone and the muscle fibres that it innervates can therefore be regarded as a functional unit, the motor unit: the arrangement accounts for the more or less simultaneous contraction of a number of fibres within the muscle. The size of a motor unit varies considerably. In muscles used for precision tasks, e.g. extraocular muscles, interossei and intrinsic laryngeal muscles, each motor neurone innervates perhaps 10 muscle fibres, whereas in a large limb muscle, a motor neurone may innervate several hundred muscle fibres. Within a muscle, the fibres belonging to one motor unit are distributed over a wide territory, without regard to fascicular boundaries, and intermingle with the fibres of other motor units. The motor units become larger in cases of nerve damage, because denervated fibres induce collateral or terminal sprouting of the remaining axons. Each new branch can reinnervate a fibre, thus increasing the territory of its parent motor neurone.
Muscle contraction: basic physiology
The arrival of an action potential at the motor end plate of a neuromuscular junction causes acetylcholine (ACh) to be released from storage vesicles into the highly infolded 30–50 nm synaptic cleft that separates the nerve ending from the sarcolemma (see Fig. 3.37). ACh is rapidly bound by receptor molecules located in the junctional folds, triggering an almost instantaneous increase in the permeability, and hence conductance, of the postsynaptic membrane. This generates a local depolarization (the end-plate potential), which initiates an action potential in the surrounding sarcolemma. The activity of the neurotransmitter is rapidly terminated by the enzyme acetylcholinesterase (AChE), which is bound to the basal lamina in the sarcolemmal junctional folds. The sarcolemma is an excitable membrane, and action potentials generated at the neuromuscular junction propagate rapidly over the entire surface of the muscle fibre.
The lengths of the thick and thin filaments do not change during muscle contraction. The sarcomere shortens by the sliding of thick and thin filaments past one another, which draws the Z-discs towards the middle of each sarcomere (Fig. 5.41). As the overlap increases, the I- and H-bands narrow to near extinction, while the width of the A-bands remains constant. Filament sliding depends on the making and breaking of bonds (cross-bridge cycling) between myosin head regions and actin filaments. Myosin heads ‘walk’ or ‘row’ along actin filaments using a series of short power strokes, each resulting in a relative movement of 5–10 nm. Actin filament binding sites for myosin are revealed only in the presence of calcium, which is released into the sarcoplasm from the sarcoplasmic reticulum, causing a repositioning of the troponin–tropomyosin complex on actin (the calcium-sensitive switch). Myosin head binding and release are both energy dependent (ATP binding is required for detachment of bound myosin heads as part of the normal cycle). In the absence of ATP (as occurs postmortem) the bound state is maintained, and is responsible for the muscle stiffness known as rigor mortis.
Slow twitch vs fast twitch
In man, all muscles are mixed; fibres that are specialized for aerobic working conditions intermingle with fibres of a more anaerobic or intermediate metabolic character. The different types of fibre are not readily distinguished in routine histological preparations but are clear when specialized enzyme histochemical techniques are used. On the basis of metabolic differences, individual fibres can be classified as predominantly oxidative, slow twitch (red) fibres, or glycolytic, fast twitch (white) fibres. Muscles composed mainly of oxidative, slow twitch fibres correspond to the red muscles of classical descriptions. Muscles that are predominantly oxidative in their metabolism contract and relax more slowly than muscles relying on glycolytic metabolism. This difference in contractile speed is due in part to the activation mechanism (volume density of sarcotubular system and proteins of the calcium ‘switch’ mechanism), and in part to molecular differences between the myosin heavy chains of these types of muscle. These differences affect the ATPase activity of the myosin head, which in turn alters the kinetics of its interaction with actin, and hence the rate of cross-bridge cycling. Differences between myosin isoforms may be detected histochemically: ATPase histochemistry continues to play a significant role in diagnostic typing (Table 5.2). Two main categories have been described: type I fibres, which are slow-contracting, and type II, which are fast-contracting. Molecular analyses have revealed that type II fibres may be further subdivided according to their content of myosin heavy-chain isoforms into types IIA, IIB and IIX (Schiaffino & Reggiani 1996). There is a correlation between categories and metabolism, and therefore with fatigue resistance, such that type I fibres are generally oxidative (slow oxidative) and resistant to fatigue, type IIA are moderately oxidative, glycolytic (fast oxidative glycolytic) and fatigue resistant, and IIB largely rely on glycolytic metabolism (fast glycolytic) and so are easily fatigued.
Table 5.2 Physiological, structural and biochemical characteristics of the major histochemical fibre types.
Fibre type transformation
The fibre type proportions in a named muscle may vary between individuals of different age or athletic ability. Fibre type grouping, where fibres with similar metabolic and contractile properties aggregate, increases after nerve damage and with age. It occurs as a result of reinnervation episodes, where denervated fibres are ‘taken over’ by a sprouting motor neurone and their type properties transformed under direction of the new motor neurone. If the nerves to fast white and slow red muscles are cut and cross-anastomosed in experimental animals, so that each muscle is reinnervated by the other’s nerve, the fast muscle becomes slower-contracting, and the slow muscle faster-contracting (Buller et al 1960). There is evidence that fibre type transformation may be a response to the patterns of impulse traffic in the nerves innervating the muscles. If fast muscles are stimulated continuously for several weeks at 10 Hz, a pattern similar to that normally experienced by slow muscles, they develop slow contractile characteristics and acquire a red appearance and a resistance to fatigue even greater than that of slow muscles.
Attachments of skeletal muscles
Tendons
Tendons (Fig. 5.45) take the form of cords or straps of round or oval cross-section, and consist of dense, regular connective tissue. They contain fascicles of type I collagen, orientated mainly parallel to the long axis, but are to some extent interwoven. The fasciculi may be conspicuous enough to give tendons a longitudinally striated appearance to the unaided eye. Tendons generally have smooth surfaces, although large tendons may be ridged longitudinally by coarse fasciculi (e.g. the osseous aspect of the angulated tendon of obturator internus). Loose connective tissue between fascicles provides a conduit for small vessels and nerves, and condenses on the surface as a sheath or epitendineum, which may contain elastin and irregularly arranged collagen fibres. The loose attachments between this sheath and the surrounding tissue present little resistance to movements of the tendon, but in situations where greater freedom of movement is required, a tendon is separated from adjacent structures by a synovial sheath.
Tendons are strongly attached to bones, both at the periosteum and through fasciculi (extrinsic collagen fibres). Tendinous attachments (entheses or osteotendinous junctions) have been broadly categorized as either fibrocartilagenous or fibrous. In fibrocartilagenous entheses, four zones of tissue have been identified: pure dense fibrous connective tissue (continuous with and indistinguishable from the tendon), uncalcified fibrocartilage, calcified fibrocartilage and bone (continuous with and indistinguishable from the rest of the bone). There are no sharp boundaries between the zones, and the proportions of each component vary between entheses (Fig. 5.46A,B,D). At fibrous entheses, which are characteristic of the shafts of long bones, the tendon is attached to bone by dense fibrous connective tissue either directly or indirectly via the periosteum (Fig. 5.46C). It has been suggested that the greater area of the skeleton to which many fibrous entheses (e.g. pronator teres, deltoid) are attached compared with fibrocartilagenous entheses (e.g. rotator cuff tendons) is important in dissipating stress. (For a review of entheses and the concept of the ‘enthesis organ’, see Benjamin et al 2006.)
Form and function in skeletal muscles
Direction of action
Although muscles differ in their internal architecture, the resultant force is directed along the line of the tendon: any forces transverse to this direction must therefore be in balance (Fig. 5.36, Fig. 5.47). In strap-like muscles, the transverse component is negligible. In fusiform, bipennate and multipennate muscles, symmetry in the arrangement of the fibres produces a balanced opposition between transverse components, whereas in asymmetrical muscles, e.g. unipennate muscles, the fibres generate an unopposed lateral component of force which is balanced by intramuscular pressure.
Force and range of contraction
The force developed by an active muscle is the summation of the tractive forces exerted by millions of cross-bridges as they work asynchronously in repeated cycles of attachment and detachment. This force depends on the amount of contractile machinery that is assembled in parallel, and therefore on the cross-sectional area of the muscle. The phrase ‘contractile machinery’ has been chosen deliberately here. Mechanically, it matters little that the myofilaments are assembled into myofibrils, the myofibrils into fibres, and the fibres into fascicles (see Fig. 5.38): the total area occupied by myofilamentous arrays determines the force. If the fibres are small, the force will be influenced only to the extent that more of the cross-sectional area will be occupied by non-contractile elements, such as endomysial connective tissue. If there are many small fascicles, the amount of perimysial connective tissue in the cross-section will increase.
The range of contraction generated by an active muscle depends on the relative motion that can take place between the overlapping arrays of thick and thin filaments in each sarcomere. In vertebrate muscle, the construction of the sarcomere sets a natural limit to the amount of shortening that can take place: the difference between the minimum overlap and the maximum overlap of the thick and thin filaments represents a shortening of about 30%. Since the sarcomeres are arranged in series, the muscle fibres shorten by the same percentage. The actual movement that takes place at the ends of the fibres will depend on the number of sarcomeres in series, i.e. it will be proportional to fibre length. By way of illustration, compare the behaviour of two muscles, fixed at one end, both having fibres parallel to the line of pull and the same cross-sectional area. If one muscle is twice as long as the other, then the force developed by each muscle will be the same, but the maximum movement produced at the free ends will be twice as much for the longer muscle. Muscles in which the fibres are predominantly parallel to the line of pull are often long and thin (strap-like): they develop rather low forces, but are capable of a large range of contraction. Where greater force is required the cross-sectional area must be increased, as occurs in a pennate construction (Fig. 5.48). Here, the fibres are set at an angle to the axis of the tendon (the angle of pennation). The range of contraction produced by such a muscle will be less than that of a strap-like muscle of the same mass, because the fibres are short and a smaller fraction of the shortening takes place in the direction of the tendon. The obliquely directed force can be resolved vectorially into two components, one acting along the axis of the tendon, and one at 90° to this. In symmetrical forms (Fig. 5.48), the transverse force is balanced by fibres on the opposite side of the tendon. The functionally significant component is the one acting along the axis of the tendon. As the lengths of the vectors show, less force is available in this direction than is developed by the fibres themselves. In practice, this loss is not very great: angles of pennation are usually less than 30°, and so the force in the direction of the tendon may be 90% or more of that in the fascicles (cos 30° = 0.87). Angulation of a set of fibres reduces both the force and range of contraction along the axis of the tendon. However, these negative consequences are outweighed by the design advantage conferred by pennation, i.e. the opportunity to extend the tendinous aponeurosis, and so increase the area available for the attachment of muscle fibres. A given mass of muscle can then be deployed as a large number of short fibres, increasing the total cross-sectional area, and hence the force, available. In a multipennate muscle, the effective cross-sectional area is larger still, and the fibres tend to be even shorter. The ‘gearing’ effect of pennation on a muscle therefore results from an internal exchange of fibre length for total fibre area: this allows much greater forces to be developed, but at the expense of a reduced range of contraction.
Muscles and movement
Actions of muscles
In the context of different movements, a given muscle may act differently, as a prime mover, antagonist, fixator or synergist. Even the same movement may involve a muscle in different ways if it is assisted or opposed by gravity. For example, in thrusting out the hand, triceps is the prime mover responsible for extending the forearm at the elbow, and the flexor antagonists are largely inactive. However, when the hand lowers a heavy object, the extensor action of the triceps is replaced by gravity, and the movement is controlled by active lengthening, i.e. eccentric contraction, of the flexors. It is important to remember that all movements take place against the background of gravity, and its influence must not be overlooked.
Development of skeletal muscle
A myogenic lineage, denoted by the expression of myogenic determination factors, can be demonstrated transiently in some cells shortly after their ingression through the primitive streak. Skeletal muscle found throughout the body is derived from this paraxial mesenchyme, which is formed from ingression at the streak and subsequently segmented into somites (see also the origin of extraocular muscles, Ch. 41).
Skeletal muscle originates from a pool of premyoblastic cells which arise in the dermatomyotome of the maturing somite and begin to differentiate into myoblasts at 4–5 weeks of gestation. By 6 weeks, cells have migrated from the dermatomyotomal compartment to form the myotome in the centre of the somite (see Fig. 44.3). These myotomal precursor cells are identified by the expression of myogenic determination factors; they will eventually differentiate within the somite to form the axial (or epaxial) musculature (erector spinae). A distinct cohort of precursor cells migrates away from the somite to invade the lateral regions of the embryo; there they form the muscles of the limbs (see Ch. 51), limb girdles and body wall (hypaxial musculature; see Fig. 44.3). Virtually all cells in the lateral half of the newly formed somite are destined to migrate in this way. Myogenic determination factors are not expressed in these cells until the muscle masses coalesce. The appearance of myotomal myoblasts, and the migration of myoblasts to the prospective limb region, occurs first in the occipital somites. Thereafter these processes follow the general craniocaudal progression of growth, differentiation and development of the embryo. The myoblastic cells from which the limb muscles develop do not arise in situ from local limb bud mesenchyme, as was once thought, but migrate from the ventrolateral border of those somites adjacent to the early limb buds.
Myogenic determination factors
The myogenic factors do not all appear at the same stage of myogenesis (Buckingham et al 2003). In the somites, Myf-5 is expressed early, before myotome formation, and is followed by expression of myogenin. MyoD is expressed relatively late together with the contractile protein genes. Myf-6 is expressed transiently in the myotome and becomes the major transcript postnatally. Whether this specific timing is important for muscle development is not yet clear. The creation of mutant mice deficient in the bHLH proteins (gene ‘knock-out’) has shown that myogenin is crucial for the development of functional skeletal muscle, and that while neither Myf-5 nor MyoD is essential to myogenic differentiation on their own, lack of both results in a failure to form skeletal muscle. In the limb bud (see Ch. 51) the pattern of expression of the bHLH genes is generally later than in the somite: Myf-5 is expressed first but transiently, followed by myogenin and MyoD, and eventually Myf-6. These differences provide evidence at the molecular level for the existence of distinct muscle cell populations in the limb and somites. It may be that the myogenic cells that migrate to the limb differ at the outset from those that form the myotome, or their properties may diverge subsequently under the influence of local epigenetic factors.
Formation of muscle fibres
In both myotomes and limb buds, myogenesis proceeds in the following way. Myoblasts become spindle-shaped and begin to express muscle-specific proteins. The mononucleate myoblasts aggregate and fuse to form multinucleate cylindrical syncytia, or myotubes, in which the nuclei are aligned in a central chain (Fig. 5.49). These primary myotubes attach at each end to the tendons and developing skeleton. The initiation of fusion does not depend on the presence of nerve fibres, since these do not penetrate muscle primordia until after the formation of primary myotubes.
At 14–15 weeks, primary myotubes are still in the majority, but by 20 weeks the secondary myotubes predominate. During weeks 16–17, tertiary myotubes appear: they are small and adhere to the secondary myotubes, with which they share a basal lamina. They become independent by 18–23 weeks, their central nuclei move to the periphery, and they contribute a further generation of myofibres. The secondary and tertiary myofibres are always smaller and more numerous than the primary myofibres. In some large muscles, higher order generations of myotubes may be formed.
Late in fetal life, a final population of myoblasts appears which will become the satellite cells of adult muscle. These normally quiescent cells lie outside the sarcolemma beneath the basal lamina (Fig. 5.49, Fig. 5.50). M-cadherin, a cell adhesion protein of possible regulatory significance, occurs at the site of contact between a satellite cell and its muscle fibre. In a young individual, there is one satellite cell for every 5–10 muscle fibre nuclei. The latter are incapable of DNA synthesis and mitosis, and satellite cells are therefore important as the sole source of additional muscle fibre nuclei during postnatal growth of muscle (to maintain the ratio of cytoplasmic volume per nucleus as fibres increase in mass). After satellite cells divide, one of the daughter cells fuses with the growing fibre, the other remains as a satellite cell capable of further rounds of division. Similar events may take place to support exercise-induced hypertrophy of adult skeletal muscle. Satellite cells provide a reservoir of myoblasts capable of initiating regeneration of an adult muscle after damage. Other stem cell populations may also be induced to begin a myogenic differentiation pathway, e.g. bone marrow stem cells and processed lipoaspirate cells (Mizuno et al 2002).
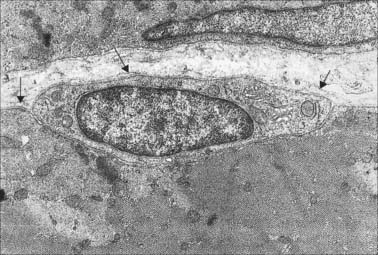
Fig. 5.50 Electron micrograph of a satellite cell. Note the two plasma membranes that separate the cytoplasm of the satellite cell from that of the muscle fibre, and the basal lamina (arrows) of the transversely-sectioned muscle fibre, which continues over the satellite cell (see also Fig. 5.38). Compare this appearance with the normal muscle nucleus which is seen in the adjacent fibre (above).
(Photograph by Dr Michael Cullen, School of Neurosciences, University of Newcastle upon Tyne.)
Growth and regulation of fibre length
Muscle fibres grow in length by addition of sarcomeres to the ends of the myofibrils. It is important that the number of sarcomeres is regulated throughout life, so that the mean sarcomere length, and hence filament overlap, is optimized for maximum force. This is achieved by addition or removal of sarcomeres in response to any prolonged change of length. For example, if a limb is immobilized in a plaster cast, the fibres of muscles that have been fixed in a shortened position lose sarcomeres, while those that have been fixed in a lengthened position add sarcomeres; the reverse process occurs after the cast has been removed.
Satellite cells and muscle repair
Studies in mouse models, where genetic analysis is possible, have shown that the functional properties of postnatal satellite cells are dependent on the expression of the Pax7 gene, whereas the prenatal development of muscle is not similarly dependent. This implies that the satellite cells are not simply the relics of the prenatal myogenic population although they appear to be derived from the same embryonic source in the somites. Moreover, satellite cells are not a homogeneous population: no two differentiation markers concur completely. This is also the situation in human tissue (Fig. 5.51). It has yet to be determined whether this variation reflects a difference in position in the lineage, in functional status, or in the adjacent environment.
The satellite cell been rigorously established in mice as being both necessary and sufficient for effective regeneration of damaged skeletal muscle. The cells proliferate to replace their resident region of muscle in 3–4 days and to replenish the quiescent satellite cell population (Collins & Partridge 2005). In man, there is histological evidence of the rapid accumulation of myoblasts, presumably derived from local satellite cells, at sites of muscle damage.
A point of wide pathological interest is the demonstration that the failing regenerative potency of satellite cells in aging muscle seems in large part to be attributable to age-related changes in the systemic environment rather than a decline in the intrinsic capabilities of the satellite cells themselves (Conboy et al 2005).