11 Essentials of Pulmonology
RESPIRATORY PROBLEMS ARE COMMON in children. The anesthesiologist often encounters pulmonary complications ranging from mild acute respiratory tract infections to chronic lung disease with end-stage respiratory failure during perioperative consultations, intraoperatively, or in the intensive care unit. This chapter discusses the basics of respiratory physiology, how to assess pulmonary function, and the practical anesthetic management of specific pulmonary problems. Airway and thoracic aspects pertinent to ventilation are discussed in Chapters 12 and 13; pulmonary issues specific to neonates, intensive care, and various disease states are addressed in the relevant chapters.
Respiratory Physiology
The morphologic development of the lung begins at several weeks after conception and continues into the first decade of postnatal life.1 Intrauterine gas exchange occurs via the placenta, but the respiratory system develops in preparation for extrauterine life, when gas exchange transfers abruptly to the lungs at birth.
Development of the lung, which begins as an outgrowth of the foregut ventral wall, can be divided into several stages (Fig. 11-1). During the embryonic period, the first few postconceptional weeks, lung buds form as a projection of the endodermal tissue into the mesenchyme. The pseudoglandular period extends to the 17th week of life, during which rapid lung growth is accompanied by formation of the bronchi and branching of the airways down to the terminal bronchioli. Further development of bronchioli and vascularization of the airways occurs during the canalicular stage of the second trimester. The saccular stage begins at approximately 24 weeks, when terminal air sacs begin to form. The capillary networks surrounding these air spaces proliferate, allowing sufficient pulmonary gas exchange for extrauterine survival of the premature neonate by 26 to 28 weeks. Formation of alveoli occurs by lengthening of the saccules and thinning of the saccular walls and has begun by the 36th postconceptional week in most human fetuses. The vast majority of alveolar formation occurs after birth, typically continuing until 8 to 10 years postnatally. The neonatal lung at birth usually contains 10 to 20 million terminal air sacs (many of which are saccules rather than alveoli), one-tenth the number in the mature adult lung. Growth of the lungs after birth occurs primarily as an increase in the number of respiratory bronchioles and alveoli rather than an increase in the size of the alveoli.
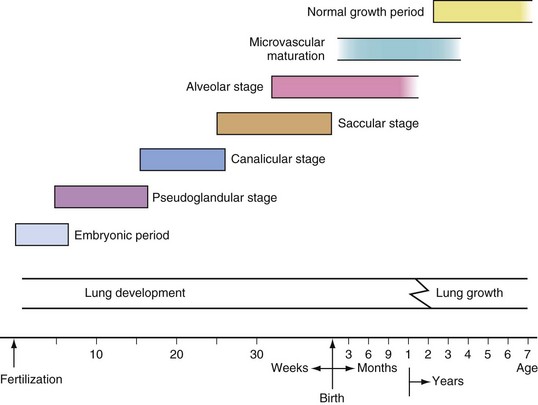
FIGURE 11-1 Timetable for lung development.
(Modified with permission from Guttentag S, Ballard PL. Lung development: embryology, growth, maturation, and developmental biology. In: Tausch HW, Ballard RA, Gleason CA, editors. Avery’s diseases of the newborn. 8th ed. Philadelphia: WB Saunders; 2004, p. 602.)
Breathing is controlled by a complex interaction involving input from sensors, integration by a central control system, and output to effector muscles.2 Afferent signaling is provided by peripheral arterial and central brainstem chemoreceptors, upper airway and intrapulmonary receptors, and chest wall and muscle mechanoreceptors.
Central integration of respiration is maintained by the brainstem (involuntary) and by cortical (voluntary) centers. Although the precise mechanism of the neural ventilatory rhythmogenesis is unknown, the pre-Bötzinger complex and the retrotrapezoid nucleus/parafacial respiratory group, neural circuits in the ventrolateral medulla, are thought to be the respiratory rhythm generators.3 These neuron groups fire in an oscillating pattern, an inherent rhythm that is moderated by inputs from other respiratory centers. Involuntary integration of sensory input occurs in various respiratory nuclei and neural complexes in the pons and medulla that modify the baseline pacemaker firing of the respiratory rhythm generators. The cerebral cortex also affects breathing rhythm and influences or overrides involuntary rhythm generation in response to conscious or subconscious activity, such as emotion, arousal, pain, speech, breath holding, and other activities.2
Preoperative Assessment
Because children may be unwilling or unable to give a reliable history, parents or caregivers are often the sole source or an important supplemental source of information during initial evaluation. Risk factors in the history that are associated with an increased risk of perioperative events include a respiratory tract infection within the preceding 2 weeks, wheezing during exercise, more than three wheezing episodes in the past 12 months, nocturnal dry cough, eczema, and a family history of asthma, rhinitis, eczema, or exposure to tobacco smoke.1,4 Viral upper respiratory tract infections (URIs) are common in children, and the time, frequency, and severity of infection should be established. If wheezing is present, the precipitating causes, frequency, severity, and relieving factors should be determined. Chronic pulmonary diseases often have a variable clinical course, and the details of acute exacerbations of chronic problems should be elicited.
Pulmonary Function Tests
Pulmonary function tests include dynamic studies, measurement of static lung volumes, and diffusing capacity. Pulmonary function tests enable clinicians to (1) establish mechanical dysfunction in children with respiratory symptoms, (2) quantify the degree of dysfunction, and (3) define the nature of the dysfunction as obstructive, restrictive, or mixed obstructive and restrictive.5 Table 11-1 presents common indications for pulmonary function testing in children.
TABLE 11-1 Uses of Pulmonary Function Studies in Children
• To establish pulmonary mechanical abnormality in children with respiratory symptoms
• To quantify the degree of dysfunction
• To define the nature of pulmonary dysfunction (obstructive, restrictive, or mixed obstructive and restrictive)
• To aid in defining the site of airway obstruction as central or peripheral
• To differentiate fixed from variable and intrathoracic from extrathoracic central airway obstruction
• To follow the course of pulmonary disease processes
• To assess the effect of therapeutic interventions and guide changes in therapy
• To detect increased airway reactivity
• To evaluate the risk of diagnostic and therapeutic procedures
• To monitor for pulmonary side effects of chemotherapy or radiation therapy
• To aid in predicting the prognosis and quantitating pulmonary disability
• To investigate the effect of acute and chronic disease processes on lung growth
Modified with permission from Castile R. Pulmonary function testing in children. In: Chernick V, Boat TF, Wilmott RW, Bush A, editors: Kendig’s disorders of the respiratory tract in children. 7th ed. Philadelphia: Elsevier Saunders; 2006, p. 168.Reproduced from National Asthma Education and Prevention Program: Full report of the expert panel: guidelines for the diagnosis and management of asthma (EPR-3). Bethesda, Md.: National Heart, Lung, and Blood Institute, National Institutes of Health; 2007.
The dynamic studies, which are the most commonly used tests, include spirometry, flow–volume loops, and measurement of peak expiratory flow. Spirometry measures the volume of air inspired and expired as a function of time and is by far the most frequently performed test of pulmonary function in children. With a forced exhalation after a maximal inhalation, the total volume exhaled is known as the forced vital capacity (FVC), and the fractional volume exhaled in the first second is known as the forced expiratory volume in 1 second (FEV1). Figure 11-2 illustrates a normal pulmonary function test (normal flow–volume loop and spirometry parameters).
An obstructive process is characterized by decreased velocity of airflow through the airways (Fig. 11-3), whereas a restrictive defect produces decreased lung volumes (Fig. 11-4). Examination of the ratio of airflow to lung volume assists in differentiating these components of lung disease. Normally, a child should be able to exhale more than 80% of the FVC in the first second. Children with obstructive lung disease have decreased airflow in relation to exhaled volume. If the volume exhaled in the first second divided by the volume of full exhalation (FEV1/FVC) is less than 80%, then airway obstruction is present (Table 11-2; see Fig. 11-3).
TABLE 11-2 Characteristics of Obstructive and Restrictive Patterns of Lung Disease
Measurement | DISEASE CATEGORY | |
---|---|---|
Obstructive | Restrictive | |
FVC | Normal/decreased | Decreased |
FEV1 | Decreased | Decreased |
FEV/FVC | Decreased | Normal |
FEV1, Forced expiratory volume in 1 second; FVC, forced vital capacity.
The FEV1 needs to be interpreted in the context of the FVC. A small FEV1 alone is insufficient evidence on which to make a diagnosis of airflow obstruction. Those with restrictive lung disease have both decreased FEV1 and FVC—decreased flow rate and reduced total exhaled volume. Restrictive lung disease is associated with a loss of lung tissue or a decrease in the lung’s ability to expand. A restrictive defect is diagnosed when the FVC is less than 80% of normal with either a normal or an increased FEV1/FVC (see Table 11-2 and Fig. 11-4).
Pulmonary function tests can also be used to differentiate fixed from variable airway obstruction and to localize the obstruction as above or below the thoracic inlet (Figs. 11-5 through 11-7, E-Fig. 11-1). This information can be gleaned from distinctive changes in the configuration of the flow–volume loop, a graphic representation of inspiratory and expiratory flow volumes plotted against time. A fixed central airway obstruction, such as a tumor or stenosis, may obstruct both inspiration and expiration, flattening the flow–volume curve on both inspiration and expiration (See Video 12-1). The child with tracheal stenosis, for example, has flattening of both inhalation and exhalation curves (see Fig. 11-6). A variable obstruction tends to affect only one part of the ventilatory cycle. On inhalation, the chest expands and draws the airways open. On exhalation, as the chest collapses, the intrathoracic airways collapse. Variable extrathoracic lesions tend to obstruct on inhalation more than exhalation, whereas variable intrathoracic lesions tend to obstruct more on exhalation. This produces the characteristic flow–volume patterns.
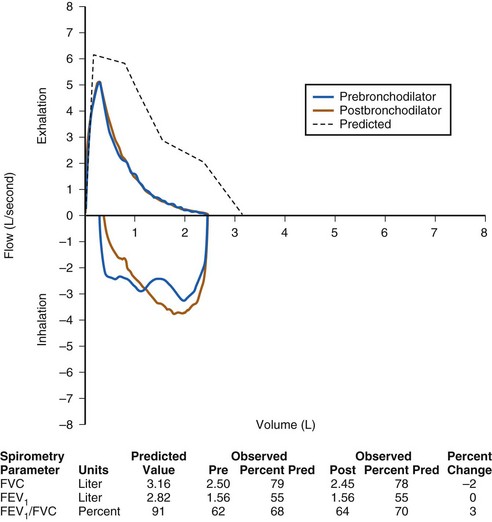
FIGURE 11-5 Pulmonary function test demonstrating a nonreversible obstructive defect. The ratio of forced expiratory volume in 1 second (FEV1) to forced vital capacity (FVC) is decreased, as is the FEV1. After administration of a short-acting bronchodilator, there is no significant improvement in the FEV1, in contrast to the pattern in Figure 11-3. This child has cystic fibrosis with a nonreversible obstructive defect. Post, Postbronchodilator; Pre, prebronchodilator; Pred, predicted value.
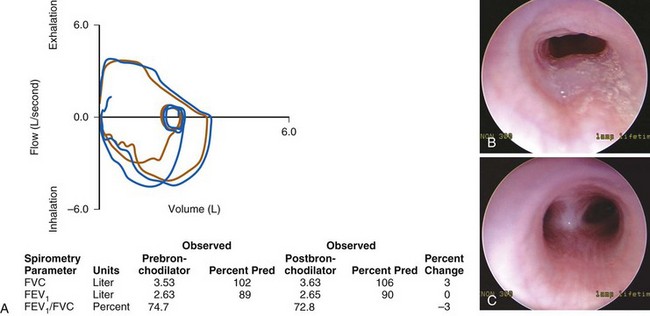
FIGURE 11-7 A, Pulmonary function test from a child with an intrathoracic airway obstruction (vascular ring). The flow–volume curves suggest a fixed expiratory obstruction. The shape of the inspiratory link is normal; the expiratory flow limb is flattened on both the prebronchodilator (brown) and postbronchodilator (blue) flow–volume curves. B, Slit-like tracheal compression before repair. C, Marked improvement in the tracheal lumen after division of the vascular ring. (See E-Fig. 11-1 for a magnetic resonance imaging angiogram of a vascular ring.)
(Photographs B and C courtesy Christopher Hartnick, MD.) FEV1, Forced expiratory volume in 1 second; FVC, forced vital capacity; Pred, predicted value.
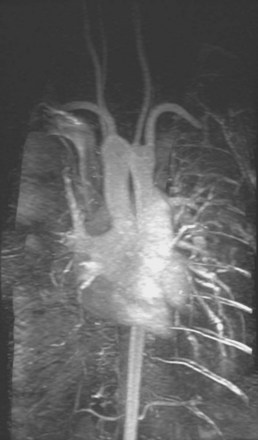
E-Figure 11-1 A magnetic resonance angiogram accompanies the flow loop in Figure 11-7, demonstrating anomalous aortic anatomy compressing the trachea.
(Courtesy Brian O’Sullivan, MD.)
In addition to diagnostic uses, spirometry is used to assess the indication for, and efficacy of, treatment. For example, the obstruction in patients with asthma is usually reversible, either gradually over time without intervention or much more rapidly after treatment with a short-acting bronchodilator. An improvement in FEV1 of 12% and 200 mL is considered a positive response. In addition to confirming the diagnosis of asthma, the degree of airflow obstruction, as indicated by the FEV1, is one measure of asthma control. A low FEV1 or an acute decrease from baseline may indicate a child whose asthma is not under good control and therefore who potentially is at greater risk for a perioperative exacerbation (see Fig. 11-3).
Perioperative Etiology and Epidemiology
Respiratory problems account for most of the perioperative morbidity in children,6,7 and cause almost one third of perioperative pediatric cardiac arrests.8 Adverse events include laryngospasm, airway obstruction, bronchospasm, hemoglobin oxygen desaturation, prolonged coughing, atelectasis, pneumonia, and respiratory failure.4,9,10 The incidence of perioperative adverse respiratory events in one study of 755 children was 34%,9 whereas in another observational study of 9297 children it was 15%.4 The triggers of these problems included airway manipulation, alteration of airway reflexes by anesthetic drugs, surgical insult, and depression of breathing caused by anesthetic and analgesic medications. Various diseases common among children can further affect the frequency of respiratory complications in pediatric anesthesia.
Studies have consistently reported greater respiratory morbidity among younger compared with older children.4,6,7,11–13 In particular, neonates are sensitive to respiratory problems for many reasons. Although the FRC approaches adult capacity (in liters per kilogram) within days after birth, a persistently large closing capacity increases the likelihood of alveolar collapse and intrapulmonary shunt. Residual patency of the ductus arteriosus can contribute to shunting. The greater metabolic rate of the infant increases oxygen requirements and decreases the time to arterial desaturation after an interruption to ventilation and gas exchange. The work of breathing is greater due to high-resistance, small-caliber airways, increased chest wall compliance, and reduced lung parenchymal compliance.
Upper Respiratory Tract Infection
Upper repiratory tract infections (URIs) are a common problem among young children. Children are typically infected several times a year, possibly even more frequently if they are in day care. Viruses cause the majority of URIs, with rhinoviruses constituting approximately one third to one half of etiologic species.14,14a Other common respiratory viruses in childhood include adenoviruses and coronaviruses.
Although most URIs are short-lived, self-limited infections and are by definition limited to the upper airway, they may increase airway sensitivity to noxious stimuli or secretions for several weeks after the infection has cleared. The mechanisms probably involve a combination of mucosal invasion, chemical mediators, and altered neurogenic reflexes.14 URIs may also impair pulmonary function by decreasing FVC, FEV1, peak expiratory flow, and diffusion capacity.15,16
Compared with uninfected children, children with a recent or current URI have an increased incidence of perioperative laryngospasm, bronchospasm, arterial hemoglobin desaturation, severe coughing, and breath holding (Table 11-3).4,12,13,17–20 However, most complications can usually be predicted and successfully managed without long-term sequelae by suitably experienced and prepared clinicians.14,18,20–23 An approach to the child with a URI is to detect the pathologic process and associated comorbidity, establish the acuteness and severity of the URI, and then decide whether to modify the anesthetic technique or postpone surgery (Table 11-4, Fig. 11-8).
TABLE 11-3 Incidence of Common Upper Respiratory Tract Infection–Associated Perioperative Adverse Events
TABLE 11-4 Risk Factors for Perioperative Adverse Events in Children with Upper Respiratory Tract Infections
For children with symptoms of an uncomplicated URI who are afebrile with clear secretions and who are otherwise healthy, anesthesia may proceed as planned, because the problems encountered are typically transient and easily managed.4,14,18,20–23 Elective surgery is usually postponed for children with more severe symptoms that include at least one of the following: mucopurulent secretions; lower respiratory tract signs (e.g., wheezing) that do not clear with a deep cough; a pyrexia greater than 100.4° F (38° C); or a change in sensorium (e.g., not behaving or playing normally, has not been eating properly).14,23
The decision to proceed with surgery becomes much more difficult when the signs of the URI are between the extremes of mild and severe. For these intermediate URIs, other considerations play a greater role in assessment of the risk/benefit ratio. These include the presence of comorbidities such as asthma, cardiac disease, or obstructive sleep apnea; a history of prematurity; the frequency of URIs; prior cancellations; the type, complexity, duration, and urgency of the surgery; the age of the child; and the socioeconomic implications for the family. The comfort level and experience of the anesthesiologist may also be an underestimated but important factor in the decision to proceed with or postpone surgery, because less experienced anesthesiologists have a greater incidence of complications.4 The need to admit a child postoperatively because of anesthetic complications or an exacerbation of the URI may expose other children to a contagious illness.
If the decision is to proceed with general anesthesia, management is directed toward avoiding stimulation of the potentially sensitized airway. Use of an endotracheal tube (ETT) should be avoided, if possible, because it increases the risk of complications, especially in younger children.4,18 Although airway management with a facemask is associated with the smallest frequency of airway complications,4 it may be inappropriate for certain cases. The laryngeal mask airway (LMA) is associated with fewer episodes of respiratory events than an ETT, but its use may similarly be contraindicated by the type of surgical procedure and the need to protect the airway from pulmonary aspiration of gastric contents.
Whichever airway technique is chosen, it is essential that the depth of anesthesia be adequate to obtund airway reflexes during placement of an airway device. The optimal depth of anesthesia at which to remove an airway device is less clearly defined. Several studies in children with and without URI did not detect a difference in emergence complications between awake and deep extubation,4,13,18,24 whereas others found a greater incidence of arterial oxygen desaturation or coughing after removal of the ETT or LMA in awake children.25,26
The optimal time when an anesthetic can be given after a URI without increasing the risk of adverse respiratory events remains contentious, but most clinicians wait 2 to 4 weeks after the resolution of the URI before proceeding.4,13,27 This reflects a balance among three critical factors: the time interval to diminish both upper and lower airway hyperreactivity; the perioperative respiratory risk, which includes a recurrence of the URI; and the need to perform the procedure.
Anesthetic techniques may affect complication rates. An observational study of 9297 children reported significantly less laryngospasm after maintenance of anesthesia with propofol than with sevoflurane.4 This finding might be attributed to a differential effect of propofol versus sevoflurane on airway reflexes.28 The effect of spraying the cords with lidocaine on the incidence of laryngospasm and bronchospasm is unclear.4 However, a randomized, controlled trial showed that topical lidocaine gel lubricant applied to the LMA in children with URIs significantly reduced the frequency of adverse airway events.29 Prophylactic treatment with glycopyrrolate, ipratropium, or albuterol is not effective in preventing URI-related adverse events.30,31 However, an observational study reported that prophylactic salbutamol was effective in reducing perioperative airway sequelae in children with URIs.32 Nasal vasoconstrictors (such as phenylephrine or oxymetazoline nose drops) have been recommended for reducing oropharyngeal secretions in children with URIs, but their efficacy remains anecdotal.23
Lower Airway Disease
Acute lower respiratory tract infections in infants and children may result in rapid deterioration necessitating aggressive intervention, including tracheal intubation and ICU admission. Many children are treated with antibiotics on the presumption that the infection is bacterial. However, many may be affected by viruses. In infants and children up to 18 months of age, respiratory syncitial virus is a very serious and common viral infection that infects the lower respiratory tract.32a Other viruses include parainfluenza virus, adenovirus, and human metapneumovirus.32b Acute inflammation of the small airways may result in bronchiolitis with edema of the small airways leading to desaturation, hypercapnia, and acute respiratory failure. In many instances, bronchiolitis results in several days of tracheal intubation until the acute infection has resolved.
Asthma is one of the most common chronic diseases of childhood, affecting by estimate more than 6 million children in the United States.33,34 A history of wheezing is associated with an increased risk of perioperative bronchospasm.4 Rare perioperative complications associated with asthma include anaphylaxis, adrenal crisis, and ventilatory barotrauma such as pneumothorax or pneumomediastinum.35 An anesthetic approach to children with asthma should include a basic understanding of the disease, an assessment of the child’s current state of health, modification of anesthetic technique as appropriate, and recognition and treatment of complications if they occur.
It is difficult to define asthma with precision, because the exact pathophysiology remains unclear. The word asthma derives from the Greek aazein, which means “to breathe with open mouth or to pant.”36 A working definition of asthma is a common chronic disorder of the airways that is complex and characterized by variable and recurring symptoms, airway obstruction, inflammation, and hyperresponsiveness of the airways.33
Clinical expressions of asthma include wheezing, chest tightness or discomfort, persistent dry cough, and dyspnea on exertion. Severe respiratory distress can occur during acute exacerbations and may be characterized by chest wall retraction, use of accessory muscles, prolonged expiration, pneumothorax, and progression to respiratory failure and death. In some children, the development of chronic inflammation may be associated with permanent airway changes, referred to as airway remodeling, that are not prevented by or fully responsive to current available treatments. There is a strong association between asthma and atopy, or immunoglobulin E (IgE)-mediated hypersensitivity.33
The diagnosis of asthma can be challenging because cough, wheezing, and bronchospasm may arise from many disease processes. Asthma itself is unlikely to be a single disease entity, and the disease process is markedly modified by various genetic and environmental factors.33,36 Many young children wheeze, and there is no definitive confirmatory blood, histologic, or radiographic diagnostic test. Given the difficulty with diagnosis, the label “preschool wheezers” may be a more appropriate description for young children with reversible airway obstruction than a diagnosis of “asthma.”36
The Tucson birth cohort study was the largest longitudinal study in the United States to attempt to differentiate wheezing or asthma phenotypes in children who did not subsequently develop asthma.37–39 This study examined 826 children at ages 3 years and 6 years from a cohort of 1246 neonates. By the age of 6 years, 48.5% of the children had experienced at least one documented episode of wheezing and were categorized into three groups. “Transient wheezers” were children who wheezed only in response to viral infections, typically during the first 3 years of life. “Non-atopic wheezers” were children who wheezed beyond the first few years of life, often in response to viral infections, but who were less likely to persistently wheeze in later childhood. “Atopy-associated wheezers” were children who had a reversible wheeze together with a tendency toward IgE-mediated hypersensitivity; they had the greatest risk of persistent symptoms into late childhood and adulthood.37
The development of asthma is a complex process that probably involves the interaction of two crucial elements: host factors (specifically genetic modifiers of inflammation) and environmental exposures (e.g., viral infections, environmental allergens, pollution) that occur during a crucial time in the development of the immune system.33 Therefore, the population of young children who wheeze includes a spectrum of disorders rather than one specific pathologic process.
Asthma must be differentiated from other distinct causes that produce similar symptoms (Table 11-5). Tracheomalacia or bronchomalacia may produce wheezing, but this tends to be present from birth (which is unusual for asthma), and the wheezing is commonly of a single pitch heard loudest in the central airways, whereas asthma typically produces polyphonic sounds from the lung periphery. Breathing difficulties due to chronic aspiration are often related to feeding times. Unremitting wheeze or stridor is often caused by a fixed obstruction or foreign body.
Acute | |
Bronchiolitis | Pneumothorax |
Asthma | Endobronchial intubation |
Foreign body | Herniated ETT cuff |
Inhalation injury | Aspiration Anaphylaxis |
Recurrent or Persistent | |
Bronchiolitis | Mediastinal mass |
Asthma | Tracheomalacia/bronchomalacia |
Foreign body | Vascular ring |
Bronchopulmonary dysplasia | |
Cardiac failure | Tracheal web/stenosis |
Cystic fibrosis | Bronchial stenosis |
Recurrent aspiration | Roundworm infestation |
Sickle cell disease |
ETT, Endotracheal tube.
Chronic cough is the most common manifestation of asthma in children. Many children who cough may never be heard to wheeze but still have asthma. A cough with or without wheeze may be caused by a viral infection, whereas a persistent, productive cough may suggest suppurative lung disease such as CF.35 A positive response of the cough to asthma medications suggests the diagnosis of asthma.
A retrospective review of 706 adult and pediatric patients with a rigorous definition of asthma found an incidence of documented bronchospasm of 1.7% and no instances of pneumonia, pneumothorax, or death.40 Of 211 children younger than 12 years of age, none developed bronchospasm at the time of surgery. A retrospective review of more than 136,000 computer-based anesthesia records found a 0.8% incidence of bronchospasm in patients with asthma.41 By contrast, older studies from the 1960s reported that 7% to 8% of asthmatic patients wheezed.42,43 A blinded, prospective study of 59 asthmatic patients detected transient wheezing after tracheal intubation in 25% of cases; however, most events were brief and self-limited.44 An observational study of 9297 children reported an overall incidence of 2% for bronchospasm; in the subgroup of 2256 children with a history of respiratory problems, the incidence was 6%.4,35 An editorial review of the subject of asthma and anesthesia concluded that, although the true incidence of major complications is small, severe adverse outcomes do result from bronchospasm, and children with asthma are at heightened risk for severe morbidity.45
Both the severity and the control of asthma must be established preoperatively. These two aspects of the current disease state should be clearly differentiated.46 For example, asthma may be severe yet well controlled, whereas even mild asthma may be poorly controlled. Both situations may present a heightened potential for perioperative complications, because even the child with intermittent but poorly controlled asthma can have a severe exacerbation.
Severity and control may be assessed by the frequency of symptoms, limitation of effort tolerance, night awakenings, medication use, emergency department attendance, hospitalizations, and need for ventilatory support. An approach to assessment of severity and control in children aged 5 to 11 years is outlined in E-Tables 11-1 through 11-3. A history of a nocturnal dry cough, more than three wheezing episodes in the past 12 months, or a history of past or present eczema is associated with an increased risk of bronchospasm.4
E-TABLE 11-1 Classification of Asthma Severity in Children 5 to 11 Years of Age Who Are Not Currently Taking Long-Term Control Medication
E-TABLE 11-2 Classification of Severity after Asthma Becomes Well Controlled, by Lowest Level of Treatment Required to Maintain Control
Maintenance treatment of asthma is based on a stepwise approach, so that the type of therapy is often an indication of severity. Short-acting inhaled β-agonists are first-line therapy, with inhaled corticosteroids for those patients with persistent symptoms poorly managed by bronchodilators as the preferred second step. Alternative treatments at this step include a leukotriene receptor antagonist, a mast cell stabilizer such as cromolyn sodium or nedocromil, and a methylxanthine bronchodilator such as theophylline. The third step in therapy involves increasing the dose of inhaled corticosteroid or adding an alternative treatment to a smaller dose of corticosteroid; a long-acting β-agonist, a leukotriene receptor antagonist, or theophylline may be considered. Step 4 involves a medium dose of corticosteroid together with a long-acting β-agonist. The final steps of therapy involve a high dose of inhaled corticosteroid or commencing an oral corticosteroid (E-Fig. 11-2).
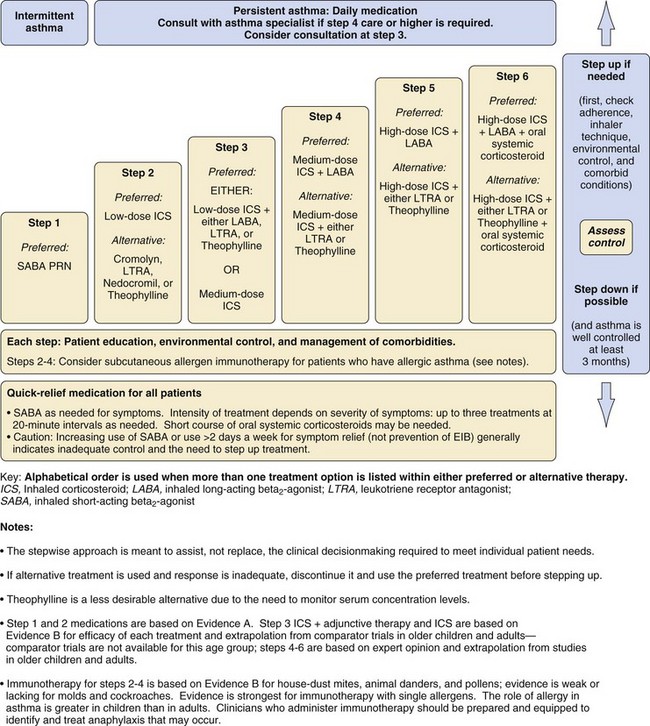
E-Figure 11-2 Stepwise approach for managing asthma in children 5 to 11 years of age.
(Reproduced from National Asthma Education and Prevention Program: Full report of the expert panel: guidelines for the diagnosis and management of asthma [EPR-3]. Bethesda, Md.: National Heart, Lung, and Blood Institute, National Institutes of Health; 2007.)
Most children with asthma have disease that is intermittent or persistent but mild and will be treated with inhaled short-acting β-agonists on an as-needed basis, alone or in combination with low-dose inhaled corticosteroids or an adjunctive therapy. Poor control may relate to poor compliance with medication, inadequate inhaler technique, or incorrect diagnosis. Severe asthma is diagnosed when symptom control is poor despite high doses of corticosteroids (steps 5 or 6 in E-Fig. 11-2). A small group of children have “brittle asthma” that is difficult to control despite optimal therapy and may lead to life-threatening respiratory compromise. A history of severe attacks or admission to intensive care is particularly ominous.
Special investigations are not routinely indicated but may be useful in specific circumstances. A chest radiograph is not usually helpful to assess the severity of asthma but can help diagnose a superimposed infection, pneumothorax, or pneumomediastinum during an acute exacerbation. Pulmonary function tests are important in monitoring long-term responses to therapy but are of little use in the immediate, routine preoperative workup of cases at a stable clinical baseline. Measurements of nitric oxide and various inflammatory markers are primarily of use as research tools at present, but their role in asthma management is evolving.47
Although an assessment of disease severity is essential, an important caveat is that many asthma deaths in the community setting occur not in those with severe disease but in those with what was thought to be mild or moderate disease. Asthma is often undertreated,46 so the sensitivity of medication prescription as a marker of disease activity must be viewed with some caution. Some studies have found a poor correlation between assessment of disease severity and the occurrence of perioperative bronchospasm. However, disease activity, as noted by recent asthma symptoms, use of medications for symptom treatment, and recent therapy in a medical facility for asthma, is significantly associated with perioperative bronchospasm.40
Children should continue their regular medications before anesthesia. Midazolam has been reported to be a safe premedication for asthmatics.48 Corticosteroids may help prevent postintubation bronchospasm in adults,49 although controlled clinical data to substantiate this practice in children are lacking.35 Inhaled β-agonists before or shortly after induction of anesthesia attenuate the increases in airway resistance associated with tracheal intubation.50,51 Ketamine is the traditional choice of intravenous induction agent in children with severe asthma, although its superiority over other agents has not been substantiated in clinical trials.52,53 Propofol is typically preferred over thiopentone because it causes less bronchoconstriction.44,54 Desflurane is associated with an increased risk of bronchospasm compared with sevoflurane or isoflurane, and because it can increase airway resistance in children, should be avoided in asthmatics.4
Tracheal stimulation is a potent stimulus for bronchospasm.4 In children with URI, in whom the airways may be acutely hyperactive, the avoidance of tracheal intubation is associated with a reduced incidence of pulmonary complications.4,17 There are inadequate clinical outcome data on the perioperative management of asthma to make definitive recommendations about airway management. Nevertheless, avoidance of tracheal and vocal cord stimulation by use of a facemask or an LMA instead of an ETT whenever possible seems a sensible approach. If tracheal intubation is mandatory, a deep plane of anesthesia is preferred to blunt airway hyperreactivity. Similarly, unless contraindicated by other factors, deep extubation is preferred for the same reason. Surgical stimulation is another trigger of bronchospasm, and anesthetic depth and analgesia should be adequate to prevent this response.
Intraoperative bronchospasm is characterized variously by polyphonic expiratory wheeze, prolonged expiration, active expiration with increased respiratory effort, increased airway pressures, a slow upslope on the end-tidal CO2 monitor, increased end-tidal CO2, and hypoxemia (Fig. 11-9). Other causes of wheezing must be excluded, such as partial obstruction of the ETT (secretions or herniation of the cuff causing obstruction), main-stem intubation (deep endobronchial intubation), aspiration, pneumothorax, or pulmonary edema. Mechanical obstruction of the circuit or ETT must also be excluded.
Inhaled β-agonists can be delivered by nebulizer or by a metered-dose inhaler down the airway device with specially designed adaptors (Fig. 11-10). Alternatively, a 60-mL syringe can be used to deliver doses of the nebulizer into the breathing circuit (E-Fig. 11-3). However, the efficiency of delivery through an inhaler that is actuated at the elbow of the breathing circuit is poor, especially in small-diameter ETTs.55 To improve the delivery efficiency of the aerosol in pediatric-size ETTs, the inhaler may be actuated 10 to 20 times at the elbow or once or twice into a narrow-gauge catheter that is passed to the end of the ETT.55,56
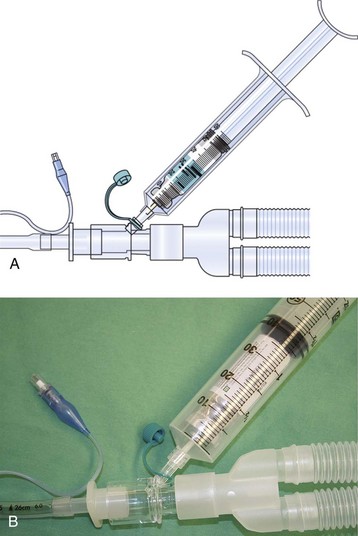
E-Figure 11-3 A and B, A 60-mL syringe may be attached to a port in the circuit to administer aerosolized drugs such as albuterol.
The anesthesiologist may be involved in the management of status asthmaticus when consulted to assist a child in the emergency department or on the wards. A drowsy, silent child with a quiet chest on auscultation is in imminent danger of respiratory arrest and requires emergent intubation by an experienced practitioner. Signs and symptoms to assess the severity of an asthma exacerbation are outlined in Table 11-6, and an algorithm for management issued by the American National Heart, Lung and Blood Institute is presented in E-Figure 11-4.
Oxygen is recommended for most children to maintain the oxygen saturation at greater than 90%. Repetitive or continuous administration of short-acting β-agonists is first-line therapy for all children and is the most effective way of reversing airflow obstruction. The addition of ipratropium to a β-agonist may produce additional bronchodilation and may have a modest effect to improve outcome. Systemic corticosteroids should be given to those who do not respond completely and promptly to β-agonists. For severe exacerbations unresponsive to the treatment listed earlier, intravenous magnesium may decrease the likelihood of intubation, although the evidence is limited. Current recommended drug doses are listed in E-Table 11-4.
There is much debate about the role of methylxanthines such as aminophylline in the management of acute exacerbations of asthma. In some countries, aminophylline is considered a first-line treatment in asthma, whereas in others it is considered second-line or used less frequently. The difference in practice may be attributed to its equivocal clinical efficacy in the treatment of acute exacerbations of asthma and to complications from toxicity (including vomiting).57–60
Children with severe atopy-associated asthma are possibly at greater risk of developing anaphylaxis in response to neuromuscular blocking drugs, antibiotics, and latex.35 Bronchospasm caused by anaphylaxis is differentiated from that due to asthma; it produces additional systemic signs such as angioedema, flushing, urticaria, and cardiovascular collapse.
Adrenal crisis during major surgical stress is a potential complication associated with severe asthma due to iatrogenic suppression of the hypothalamic-pituitary-adrenal axis.35 Adrenal suppression should be considered in any child who is taking significant doses of corticosteroids for a prolonged period. Short courses of prednisolone used to treat acute flares of asthma may affect function for up to 10 days, but prolonged dysfunction is unlikely. Large doses, prolonged therapy for more than a few weeks, and evening dosing may suppress adrenal function for up to 1 year. Prophylactic corticosteroid administration may be indicated for those receiving prolonged systemic corticosteroids, when their corticosteroid regimen is interrupted by the surgical schedule, or for those who have received high-dose inhaled corticosteroids in the recent past (see Chapter 25).
Cystic Fibrosis
CF is an autosomal recessive disorder that is caused by one of more than 1200 mutations in the gene coding for the CF transmembrane conductance regulator (located on chromosome 7), a protein that contributes to the regulation of chloride and other ion fluxes at various epithelial surfaces.61,62 The incidence of CF is approximately 1 of every 2000 births in Caucasian, making it the most common fatal inherited disease in this population.
Lung disease is the main cause of morbidity and mortality in CF, and consequently it is the focus of anesthetic concern. The pathophysiology involves mucus plugging, chronic infection, inflammation, and epithelial injury.63–65 Mucus clearance defends the lung against inhaled bacteria. The mucociliary transport system requires two fully functioning layers to be effective. The base layer of ciliary epithelia bathed in a watery liquid (sol) is overlaid by a more viscous gel (mucus) that is responsible for transporting particles along the tips of the cilia. Normally, mucus is transported at about 10 mm/min, expelling foreign particles and pathogens from the lungs. The efficacy of clearance depends on adequate hydration of the mucus.66 Lack of regulation of sodium absorption and chloride secretion decreases liquid on the airway luminal surfaces, slows mucus clearance, and promotes the formation of adherent plugs in the airway.67 Increased secretions, viscous mucus, and impaired ciliary clearance contribute to airway impaction, providing a nidus for infection.
At birth, the lung structure is almost normal.61 However, chronic and recurrent bacterial infections occur early in life, assisted by the pooling of secretions and impaired neutrophil bacterial killing on airway surfaces.63,68 Repeated and persistent infections stimulate a chronic neutrophilic inflammatory response, ultimately destroying the airway walls. Early pathogens include Staphylococcus aureus and Haemophilus influenzae. Pseudomonas aeruginosa typically invade later in life, acquire a mucoid phenotype, and form a biofilm in the lung, an event that is associated with accelerated decline in pulmonary function. The invasion of the lung by antibiotic-resistant pathogens such as certain strains of Burkholderia cepacia is often devastating, markedly increasing the death rate from lung disease.
Various insults such as bacteria, viruses, and airborne irritants can cause acute exacerbations of respiratory symptoms of cough and sputum production. This is often accompanied by systemic manifestations such as weight loss, anorexia, and fatigue. These changes from baseline are termed pulmonary exacerbations.69
Pulmonary function abnormalities are commonly obstructive in nature and include increased FRC, decreased FEV1, decreased peak expiratory flow rate, and decreased vital capacity (see Fig. 11-5). Compensatory hyperventilation typically produces a reduced Paco2, although hypercapnia may supersede in end-stage pulmonary pathology. End-stage cor pulmonale may lead to cardiomegaly, fluid retention, and hepatomegaly.
Malnutrition is a common problem in CF that follows from pancreatic insufficiency, failure of enzyme secretion, impaired gastrointestinal motility, abnormal enterohepatic circulation of bile, increased caloric demand due to severe lung disease, and anorexia of chronic disease.61 Low weight and body mass index are closely associated with, and can predict, poor lung function.
CF-related diabetes arises from progressive pancreatic disease and scarring that compromises the pancreatic islets. More than 12% of CF teenagers older than 13 years of age have insulin-dependent diabetes, and the incidence increases with age. Evidence is accumulating that diabetes contributes to the lung disease and worse outcome.63–65 In addition, classic diabetic complications occur in older CF patients. Hepatic dysfunction decreases plasma cholinesterase and clotting factors II, VII, IX, and X, whereas malabsorption of vitamin K may also contribute to coagulation issues.70
When CF was first distinguished from celiac syndrome in 1938, life expectancy was approximately 6 months. Since then, substantial advances in sustained multidisciplinary supportive care have increased the median survival time to 35 years (E-Fig. 11-5).61,71 Currently almost half of the CF population are adults.70 The pillars of treatment include nutritional repletion, relief of airway obstruction, and antibiotic therapy for lung infection. Suppression of inflammation has been a more recent focus of therapy. Organ transplantation, and in particular lung transplantation, has been used in an attempt to improve quality of life and prolong survival, but a clear benefit remains to be demonstrated.71
The multisystem nature of the disease and changing demographics mean that children present for a wide variety of surgical procedures. The most common indications for anesthesia in children are nasal polypectomy and ear, nose, and throat surgery, as a result of the frequency of upper airway pathologic processes such as chronic sinusitis and nasal polyps (Table 11-7).72,73 The investigation or correction of gastrointestinal disorders is the next most common procedural category that requires anesthesia in the CF population. Other indications for anesthesia include bronchoscopy and pulmonary lavage, gastrointestinal endoscopy, sclerosing injection of varices due to portal hypertension, insertion of venous access devices, and incidental surgical problems.73–75
TABLE 11-7 Most Frequent Indications for Anesthesia in Cystic Fibrosis
Neonates | Children/Teenagers | Adults |
---|---|---|
Meconium ileus | Nasal polypectomy | Esophageal varices |
Meconium peritonitis | Intravenous access | Recurrent pneumothorax |
Intestinal atresia | Ear/nose/throat surgery | Cholecystectomy |
Lung (liver) transplantation |
Modified from Della Rocca G. Anaesthesia in patients with cystic fibrosis. Curr Opin Anaesthesiol 2002;15:95-101.
Because of the increasing longevity of this population, the pediatric anesthesiologist may also be involved in the care of adults.70 Surgical procedures in adults typically include treatment of recurrent pneumothorax, cholecystectomy, and lung or cardiac transplantation. Consultation may also be requested for obstetric cases as increasing numbers of patients survive to adulthood.
Pulmonary disease is the predominant concern when planning anesthesia for these patients. Historically, morbidity and mortality from pulmonary complications were significant, but more recently, the mortality rate has decreased. In 1964, a retrospective study reported a perioperative mortality rate of 27%,76 but by 1972, this incidence had decreased to 4%.72 More recent studies confirmed a substantial morbidity rate with a low mortality rate. An observational study of 333 anesthesias for bronchoalveolar lavage reported minor complications (transient worsening of cough or low-grade postoperative fever or both) in 52% of patients; more serious problems, including prolonged intraoperative oxygen desaturation or high fever, occurred in 12% of children.77,78 A report of 45 bronchoscopies under anesthesia noted a rate of minor intraoperative complications (coughing, desaturation) of 13.3%.79 A study of 199 anesthesias in 53 patients for ear/nose/throat surgery reported a 5% incidence of minor pulmonary problems and no deaths.80 A report of 126 anesthesias found no mortality and a CF-specific complication rate of 9% (predominantly pulmonary complications).73 A 1984 study of 18 patients undergoing anesthesia for pleural surgery concluded that, although the risks for this procedure were great, the anesthetic hazards of CF could be minimized with careful management.81 In 11 patients undergoing anesthesia for injection of esophageal varices, there were no serious anesthetic complications, but significant deteriorations in pulmonary function tests were detected 48 hours after general anesthesia.82 It was unclear if these changes persisted past the immediate postoperative period. A larger study found no difference between pulmonary function tests measured 3 months before and 3 months after surgery.73 Although pulmonary morbidity is a significant problem, mortality is infrequent with modern anesthetic management techniques.
An assessment of the severity, current state, and progression of pulmonary disease should guide anesthetic planning. Fitness is a positive predictor of survival,61 and exercise tolerance is a useful marker of pulmonary function. The quality and quantity of secretions, recent and chronic infections, use and effectiveness of bronchodilators, and number of hospitalizations are also important points to elucidate in the history. Examination of the cardiopulmonary systems should aim to detect compromise of cardiac, pulmonary, and hepatic function. Special investigations are not routinely indicated but may quantify organ dysfunction in end stages of the disease. Arterial blood gas analysis, chest radiography, pulmonary function tests, electrocardiography, echocardiography, and liver function tests may assist the planning of anesthetic technique in selected children.75
Children are often emotionally vulnerable, not simply because of the usual preoperative anxieties but because of the psychological consequences of progression of an ultimately fatal disease. A preoperative visit should aim to allay distress; oral benzodiazepines have been successfully used as anxiolytics.73,80 Prophylactic use of osmotic laxatives may be indicated if opioid-induced ileus is anticipated.75
Because dehydration of secretions is a central pulmonary issue in CF, general anesthesia poses specific problems. During spontaneous ventilation under normal conditions, inspired gases are warmed to body temperature and saturated with water vapor, reaching this state at the isothermic saturation point just distal to the carina.83,84 This ensures that the lower airways are kept moist and warm. The alveolar environment in optimal circumstances has a saturated water vapor pressure of 47.1 mm Hg and an absolute humidity of 43.4 g/m−3 at 98.6° F (37° C).
The inspiration of cold, desiccated anesthetic gases and vapors can impair the warming and humidification of the airways. The use of any airway device (oropharyngeal airway, laryngeal mask, or ETT) bypasses the nasal and oropharyngeal passages and delivers cold, dry gas farther down the airway.85 This shifts the isothermic saturation point distally, forcing bronchi that normally function in optimal conditions to take part in heat and gas exchange.84 These parts of the airway are less adapted to moisture exchange and tend to dehydrate more rapidly, thereby impairing the mucociliary escalator and predisposing to impaction of secretions.86,87 Direct impairment of mucociliary motion by anesthetic medications, as well as blunting of the cough response and ventilatory drive, can further contribute to the problem.
It is therefore particularly important to minimize mucus desiccation in the perioperative period. Inhalation of hypertonic saline (7% sodium chloride) accelerates mucus clearance, increases lung function, and improves quality of life88–90; this is now typically part of the routine maintenance management of CF. Nebulized saline treatments should continue up to the start of anesthesia and recommence after the procedure. Inhaled gases should be humidified, or an artificial “nose” should be inserted into the circuit to conserve airway moisture and minimize inspissation of secretions.
Although removal of pulmonary secretions is considered important in principle, a small prospective trial of intraoperative bronchial wash-out and physical therapy reported an acute increase in airway resistance with no significant long-term benefit in measures of lung function.91
Sickle Cell Disease
SCD is an inherited hemoglobinopathy that results from a point mutation on chromosome 11. The mutant gene codes for the production of hemoglobin S, a mutant variant of the normal hemoglobin A. This leads to widespread and progressive vascular damage.92,93 Clinical features of the disease include acute episodes of pain, acute and chronic pulmonary disease, hemorrhagic and occlusive stroke, renal insufficiency, and splenic infarction, with mean life expectancy shortened to just over 3 decades.94 Perioperative problems and management are covered in more detail in Chapter 9, and the discussion here is limited to a brief review of the pulmonary pathology of SCD.
Acute chest syndrome (ACS) is an acute lung injury caused by SCD. Diagnostic criteria include a new pulmonary infiltrate involving at least one lung segment on the radiograph (excluding atelectasis) combined with one or more symptoms or signs of chest pain, pyrexia greater than 101.3° F (38.5° C), tachypnea, wheezing, or cough.95–97 Precipitants include infection, fat embolism after bone marrow infarction, pulmonary infarction, and surgical procedures.97–99 Potential risk factors for the development and severity of perioperative ACS include a history of lung disease, recent clustering of acute pulmonary complications, pregnancy, increased age, and the invasiveness of the surgical procedure.92 A study of 60 laparoscopic surgeries noted that ACS was associated with younger-age patients, reduced body temperature, and greater blood loss.100
Minor procedures such as inguinal hernia repair or distal extremity surgery have a reduced risk of pulmonary complications (<5%), whereas intraabdominal or major joint surgery has an ACS rate of 10% to 15%.99,101,102 Although the overall perioperative mortality from SCD is quite small, <1%,99 ACS can lead to prolonged postoperative hospitalization, respiratory failure, and death. One study of 604 patients noted that ACS typically developed about 3 days postoperatively and persisted for approximately 8 days; 2 patients died in approximately 60 episodes.98
SCD also causes chronic lung damage, known as sickle cell lung disease (SCLD).103 Because lung function has not yet been assessed longitudinally in a cohort from early childhood to adulthood, the precise pathology of and relationship between the obstructive and restrictive patterns of lung disease is unclear.104 Children appear to have a predominantly obstructive pattern,105 whereas adults have more restrictive pulmonary findings.103,106,107 The later stages of lung damage involve decreased vital and total lung capacities, impaired gas diffusion, pulmonary fibrosis, pulmonary artery hypertension, right-sided cardiomyopathy, and progressive hypoxemia.103,107 The development of pulmonary artery hypertension, which can precede clinically apparent lung damage, is a particularly ominous sign of disease progression and is associated with a heightened risk of sudden death.106 Recurrent ACS is an independent risk factor for the development of end-stage SCLD, but subtle evidence of parenchymal and vascular damage commonly precedes clustered episodes of ACS.103
Assessment of lung function should include a history of the occurrence, frequency, severity, and known precipitants of ACS and a search for progression of chronic lung damage. A recent chest radiograph can serve as a baseline for comparison if postoperative radiographs are needed and can also delineate lung pathology. Early features of lung damage include decreased distal pulmonary vascularity and diffuse interstitial fibrosis, whereas later stages are characterized by pulmonary fibrosis, pulmonary hypertension, and right ventricular hypertrophy.103,108 Pulmonary function testing can reveal the need for bronchodilators and the presence of obstructive or restrictive lung disease.
The efficacy of preoperative or intraoperative management techniques beyond basic standards of care has not been clearly demonstrated; well-delivered anesthetic and postoperative care may be the best guarantor of a good outcome.92,93 Because the effect of perioperative red blood cell transfusion (versus no transfusion) in preventing ACS or other SCD complications has not been tested by an adequately controlled study, the efficacy of prophylactic erythrocyte transfusion is controversial. One guideline suggested that children in low-risk situations should not be transfused prophylactically, but children in high-risk situations may be transfused.92 A survey of North American pediatric anesthesiologists found that most tend not to transfuse children assessed as being at low risk undergoing minor procedures, whereas a greater number do transfuse those at greater risk (i.e., sicker children undergoing more invasive procedures).109
If transfusion is undertaken, exchange transfusion aiming to decrease the concentration of hemoglobin S to 30% is no more efficacious than correction of anemia to a hematocrit of 30% in preventing SCD exacerbations but results in more transfusion-related complications.98 Consequently, if a decision is made to transfuse in the hope of preventing ACS, the target should be a hematocrit of 30% rather than a specific dilution of hemoglobin S.
The one high-risk group that merits mention are children who have experienced or are at risk for a stroke. Risk factors for strokes include low hemoglobin, hypertension, and male gender as well as three single nucleotide polymorphisms.110,111 Transcranial Doppler ultrasound and magnetic resonance imaging of the brain have been used serially to detect pathologic changes in blood flow or subclinical strokes, respectively, and in these children transfusion has been effective in reducing subsequent strokes.112,113 Silent cerebral strokes have been detected in up to 30% of asymptomatic children with SCD.110 To reduce the risk of stroke, these children are transfused at regular intervals, based on the results of the serial investigations. However, this approach raises concern about iron overload and other complications associated with repeated blood transfusions. A recent study to limit the number of transfusions in those at risk for a stroke had to be stopped prematurely because two strokes occurred despite serial transcranial Doppler monitoring.114 Chronic hydroxyurea therapy has also been shown to be effective in reducing the risk of stroke.115
Children with SCD frequently develop postoperative atelectasis. It is unclear whether this relates to an underlying sickle lung disease, difficulty with analgesia, other causes, or a combination of factors. Pain management can be difficult in these children. Large doses of opioids can depress ventilation and cause atelectasis.116 ACS tends to involve the lower segments of the lung,97 suggesting an association between atelectasis and ACS. Incentive spirometry can prevent the development of atelectasis and pulmonary infiltrates associated with ACS.117 Regional analgesia, supplemental nonopioid analgesics, prophylactic incentive spirometry, early mobilization, and good pulmonary toilet may decrease the incidence of atelectasis and ACS.
Treatment of ACS is focused on supporting gas exchange. Supplemental oxygen, noninvasive ventilatory support such as continuous positive airway pressure, or intubation and mechanical ventilation are indicated by the degree of dysfunction. Bronchodilators, incentive spirometry, and chest physiotherapy may be useful in preventing progression of the disease. In the presence of a significant ventilation/perfusion mismatch, correction of anemia can improve arterial oxygenation. Erythrocyte transfusion increases oxygen-carrying capacity, decreases fractional peripheral tissue extraction, and increases returning venous oxygen levels. Because the mean arterial oxygen content in the presence of a shunt is significantly affected by the oxygenation of blood returning from nonventilated parts of the lung, increasing venous oxygen levels can improve arterial oxygen content. Although transfusion has not been clearly shown to improve outcome, both exchange and simple transfusion can improve oxygenation.97
Bishop MJ, Cheney FW. Anesthesia for patients with asthma: low risk but not no risk. Anesthesiology. 1996;85:455–456.
A thoughtful editorial on the implications, dangers, and practical implications of asthma.
Davis PB. Cystic fibrosis since 1938. Am J Respir Crit Care Med. 2006;173:475–482.
A succinct discourse on the evolution of management of cystic fibrosis.
Firth PG, Head CA. Sickle cell disease and anesthesia. Anesthesiology. 2004;101:766–785.
A comprehensive review of anesthetic management of sickle cell disease.
Huffmeyr JL, Littlewood KE, Nemergut EC. Perioperative management of the adult with cystic fibrosis. Anesth Analg. 2009;109:1949–1961.
An updated review of anesthetic implications of advanced cystic fibrosis.
National Asthma Education and Prevention Program. Full report of the expert panel: guidelines for the diagnosis and management of asthma (EPR-3). Bethesda, Md.: National Heart, Lung, and Blood Institute, National Institutes of Health; 2007.
An extensive review of current evidence on the pathophysiology, diagnosis, and management of asthma.
Tait AR, Malviya S. Anesthesia for the child with an upper respiratory tract infection: still a dilemma? Anesth Analg. 2005;100:59–65.
von Ungern-Sternberg BS, Boda K, Chambers NA, et al. Risk assessment for respiratory complications in paediatric anaesthesia: a prospective cohort study. Lancet. 2010;376:773–783.
1 Sadler TW. Respiratory system. In: Sadler TW, ed. Langman’s medical embryology. 8th ed. Philadelphia: Lippincott Williams & Wilkins; 2000:260–269.
2 Caruana-Montaldo B, Gleeson K, Zwillich CW. The control of breathing in clinical practice. Chest. 2000;117:205–225.
3 Feldman JL, Del Negro CA. Looking for inspiration: new perspectives on respiratory rhythm. Nat Rev Neurosci. 2006;7:232–242.
4 von Ungern-Sternberg BS, Boda K, Chambers NA, et al. Risk assessment for respiratory complications in paediatric anaesthesia: a prospective cohort study. Lancet. 2010;376:773–783.
5 Castile R, Davis SD. Pulmonary function testing in children. In ’Chernick V, Boat T, Wilmott R, et al, eds.: Kendig’s disorders of the respiratory tract in children, 8th ed, Philadelphia: WB Saunders, 2011.
6 Tay CL, Tan GM, Ng SB. Critical incidents in paediatric anaesthesia: an audit of 10 000 anaesthetics in Singapore. Paediatr Anaesth. 2001;11:711–718.
7 Murat I, Constant I, Maud’huy H. Perioperative anaesthetic morbidity in children: a database of 24,165 anaesthetics over a 30-month period. Paediatr Anaesth. 2004;14:158–166.
8 Bhananker SM, Ramamoorthy C, Geiduschek JM, et al. Anesthesia-related cardiac arrest in children: update from the Pediatric Perioperative Cardiac Arrest Registry. Anesth Analg. 2007;105:344–350.
9 Mamie C, Habre W, Delhumeau C, Argiroffo CB, Morabia A. Incidence and risk factors of perioperative respiratory adverse events in children undergoing elective surgery. Paediatr Anaesth. 2004;14:218–224.
10 Rock P, Rich PB. Postoperative pulmonary complications. Curr Opin Anaesthesiol. 2003;16:123–131.
11 Cohen MM, Cameron CB, Duncan PG. Pediatric anesthesia morbidity and mortality in the perioperative period. Anesth Analg. 1990;70:160–167.
12 Bordet F, Allaouchiche B, Lansiaux S, et al. Risk factors for airway complications during general anaesthesia in paediatric patients. Paediatr Anaesth. 2002;12:762–769.
13 von Ungern-Sternberg BS, Boda K, Schwab C, et al. Laryngeal mask airway is associated with an increased incidence of adverse respiratory events in children with recent upper respiratory tract infections. Anesthesiology. 2007;107:714–719.
14 Tait AR, Malviya S. Anesthesia for the child with an upper respiratory tract infection: still a dilemma? Anesth Analg. 2005;100:59–65.
14a Greenberg SB. Respiratory consequences of rhinovirus infection. Arch Internal Med. 2003;163:278–284.
15 Collier AM, Pimmel RL, Hasselblad V, et al. Spirometric changes in normal children with upper respiratory infections. Am Rev Respir Dis. 1978;117:47–53.
16 Dueck R, Prutow R, Richman D. Effect of parainfluenza infection on gas exchange and FRC response to anesthesia in sheep. Anesthesiology. 1991;74:1044–1051.
17 Parnis SJ, Barker DS, van der Walt JH. Clinical predictors of anaesthetic complications in children with respiratory tract infections. Paediatr Anaesth. 2001;11:29–40.
18 Tait AR, Malviya S, Voepel-Lewis T, et al. Risk factors for perioperative adverse respiratory events in children with upper respiratory tract infections. Anesthesiology. 2001;95:299–306.
19 Kinouchi K, Tanigami H, Tashiro C, et al. Duration of apnea in anesthetized infants and children required for desaturation of hemoglobin to 95%: the influence of upper respiratory infection. Anesthesiology. 1992;77:1105–1107.
20 Rolf N, Coté CJ. Frequency and severity of desaturation events during general anesthesia in children with and without upper respiratory infections. J Clin Anesth. 1992;4:200–203.
21 Lewis IH. Cancelling children for elective surgery with respiratory tract infections. Bull R Coll Anaesth. 2001;6:260–264.
22 Coté CJ. The upper respiratory tract infection (URI) dilemma: fear of a complication or litigation? Anesthesiology. 2001;95:283–285.
23 Lerman J. Perioperative respiratory complications in children. Lancet. 2010;376:745–746.
24 Patel RI, Hannallah RS, Norden J, Casey WF, Verghese ST. Emergence airway complications in children: a comparison of tracheal extubation in awake and deeply anesthetized patients. Anesth Analg. 1991;73:266–270.
25 Pounder DR, Blackstock D, Steward DJ. Tracheal extubation in children: halothane versus isoflurane, anesthetized versus awake. Anesthesiology. 1991;74:653–655.
26 Kitching AJ, Walpole AR, Blogg CE. Removal of the laryngeal mask airway in children: anaesthetized compared with awake. Br J Anaesth. 1996;76:874–876.
27 Tait AR. Anesthetic management of the child with an upper respiratory tract infection. Curr Opin Anaesthesiol. 2005;18:603–607.
28 Oberer C, von Ungern-Sternberg BS, Frei FJ, Erb TO. Respiratory reflex responses of the larynx differ between sevoflurane and propofol in pediatric patients. Anesthesiology. 2005;103:1142–1148.
29 Schebesta K, Guloglu E, Chiari A, Mayer N, Kimberger O. Topical lidocaine reduces the risk of perioperative airway complications in children with upper respiratory tract infections. Can J Anaesth. 2010;57:745–750.
30 Tait AR, Burke C, Voepel-Lewis T, et al. Glycopyrrolate does not reduce the incidence of perioperative adverse events in children with upper respiratory tract infections. Anesth Analg. 2007;104:265–270.
31 Elwood T, Morris W, Martin LD, et al. Bronchodilator premedication does not decrease respiratory adverse events in pediatric general anesthesia. Can J Anaesth. 2003;50:277–284.
32 von Ungern-Sternberg BS, Habre W, Erb TO, Heaney M. Salbutamol premedication in children with a recent respiratory tract infection. Paediatr Anaesth. 2009;19:1064–1069.
32a Bezerra PGM, Britto MCA, Correia JB, et al. Viral and atypical bacterial detection in acute respiratory infection in children under five years. PLOS ONE. 2011;6:e18928.
32b Pavia AT. Viral infections of the lower respiratory tract: old viruses, new viruses, and the role of diagnosis. Clinical Infectious Diseases. 2011;52(Suppl 4):S284–S289.
33 National Heart, Lung, and Blood Institute, National Asthma Education and Prevention Program. Expert panel report 3: guidelines for the diagnosis and management of asthma. http://www.nhlbi.nih.gov/guidelines/asthma/asthgdln.pdf, 2007. Available at (accessed May 2012)
34 Akinbami LJ, Moorman JE, Garbe PL, Sondik EJ. Status of childhood asthma in the United States, 1980-2007. Pediatrics. 2009;123(Suppl 3):S131–S145.
35 Doherty GM, Chisakuta A, Crean P, Shields MD. Anesthesia and the child with asthma. Paediatr Anaesth. 2005;15:446–454.
36 A plea to abandon asthma as a disease concept. Lancet. 2006;368:705.
37 Martinez FD. Development of wheezing disorders and asthma in preschool children. Pediatrics. 2002;109:362–367.
38 Martinez FD. What have we learned from the Tucson Children’s Respiratory Study? Paediatr Respir Rev. 2002;3:193–197.
39 Stein RT, Holberg CJ, Morgan WJ, et al. Peak flow variability, methacholine responsiveness and atopy as markers for detecting different wheezing phenotypes in childhood. Thorax. 1997;52:946–952.
40 Warner DO, Warner MA, Barnes RD, et al. Perioperative respiratory complications in patients with asthma. Anesthesiology. 1996;85:460–467.
41 Empey DW, Laitinen LA, Jacobs L, Gold WM, Nadel JA. Mechanisms of bronchial hyperreactivity in normal subjects after upper respiratory tract infection. Am Rev Respir Dis. 1976;113:131–139.
42 Gold MI, Helrich M. A study of complications related to anesthesia in asthmatic patients. Anesth Analg. 1963;42:238–293.
43 Shnider SM, Papper EM. Anesthesia for the asthmatic patient. Anesthesiology. 1961;22:886–892.
44 Pizov R, Brown RH, Weiss YS, et al. Wheezing during induction of general anesthesia in patients with and without asthma: a randomized, blinded trial. Anesthesiology. 1995;82:1111–1116.
45 Bishop MJ, Cheney FW. Anesthesia for patients with asthma: low risk but not no risk. Anesthesiology. 1996;85:455–456.
46 O’Byrne PM, Parameswaran K. Pharmacological management of mild or moderate persistent asthma. Lancet. 2006;368:794–803.
47 Barnes PJ, Dweik RA, Gelb AF, et al. Exhaled nitric oxide in pulmonary diseases: a comprehensive review. Chest. 2010;138:682–692.
48 Kil N, Zhu JF, VanWagnen C, Abdulhamid I. The effects of midazolam on pediatric patients with asthma. Pediatr Dent. 2003;25:137–142.
49 Silvanus MT, Groeben H, Peters J. Corticosteroids and inhaled salbutamol in patients with reversible airway obstruction markedly decrease the incidence of bronchospasm after tracheal intubation. Anesthesiology. 2004;100:1052–1057.
50 Scalfaro P, Sly PD, Sims C, Habre W. Salbutamol prevents the increase of respiratory resistance caused by tracheal intubation during sevoflurane anesthesia in asthmatic children. Anesth Analg. 2001;93:898–902.
51 Wu RS, Wu KC, Wong TK, et al. Effects of fenoterol and ipratropium on respiratory resistance of asthmatics after tracheal intubation. Br J Anaesth. 2000;84:358–362.
52 Howton JC, Rose J, Duffy S, Zoltanski T, Levitt MA. Randomized, double-blind, placebo-controlled trial of intravenous ketamine in acute asthma. Ann Emerg Med. 1996;27:170–175.
53 Youssef-Ahmed MZ, Silver P, Nimkoff L, Sagy M. Continuous infusion of ketamine in mechanically ventilated children with refractory bronchospasm. Intensive Care Med. 1996;22:972–976.
54 Wu RS, Wu KC, Sum DC, Bishop MJ. Comparative effects of thiopentone and propofol on respiratory resistance after tracheal intubation. Br J Anaesth. 1996;77:735–738.
55 Taylor RH, Lerman J. High-efficiency delivery of salbutamol with a metered-dose inhaler in narrow tracheal tubes and catheters. Anesthesiology. 1991;74:360–363.
56 Shorten G, Dolovich M, Kuang A, et al. Safety and efficiency of metered dose inhaler delivery of salbutamol in the intubated rabbit. Crit Care Med. 2000;28:1055–1058.
57 Mitra A, Bassler D, Ducharme FM. Intravenous aminophylline for acute severe asthma in children over 2 years using inhaled bronchodilators. Cochrane Database Syst Rev. 2001. CD001276
58 D’ ’Avila RS, Piva JP, Marostica PJ, Amantea SL. Early administration of two intravenous bolus of aminophylline added to the standard treatment of children with acute asthma. Respir Med. 2008;102:156–161.
59 Babl FE, Sheriff N, Borland M, et al. Paediatric acute asthma management in Australia and New Zealand: practice patterns in the context of clinical practice guidelines. Arch Dis Child. 2008;93:307–312.
60 Wang XF, Hong JG. Management of severe asthma exacerbation in children. World J Pediatr. 2011;7:293–301.
61 Davis PB. Cystic fibrosis since 1938. Am J Respir Crit Care Med. 2006;173:475–482.
62 Rowe SM, Miller S, Sorscher EJ. Cystic fibrosis. N Engl J Med. 2005;352:1992–2001.
63 Accurso FJ. Update in cystic fibrosis 2005. Am J Respir Crit Care Med. 2006;173:944–947.
64 Accurso FJ. Update in cystic fibrosis 2007. Am J Respir Crit Care Med. 2008;177:1058–1061.
65 Accurso FJ. Update in cystic fibrosis 2006. Am J Respir Crit Care Med. 2007;175:754–757.
66 Randell SH, Boucher RC. Effective mucus clearance is essential for respiratory health. Am J Respir Cell Mol Biol. 2006;35:20–28.
67 Matsui H, Grubb BR, Tarran R, et al. Evidence for periciliary liquid layer depletion, not abnormal ion composition, in the pathogenesis of cystic fibrosis airways disease. Cell. 1998;95:1005–1015.
68 Moraes TJ, Plumb J, Martin R, et al. Abnormalities in the pulmonary innate immune system in cystic fibrosis. Am J Respir Cell Mol Biol. 2006;34:364–374.
69 Ferkol T, Rosenfeld M, Milla CE. Cystic fibrosis pulmonary exacerbations. J Pediatr. 2006;148:259–264.
70 Huffmyer JL, Littlewood KE, Nemergut EC. Perioperative management of the adult with cystic fibrosis. Anesth Analg. 2009;109:1949–1961.
71 Liou TG, Adler FR, Cox DR, Cahill BC. Lung transplantation and survival in children with cystic fibrosis. N Engl J Med. 2007;357:2143–2152.
72 Doershuk CF, Reyes AL, Regan AG, Matthews LW. Anesthesia and surgery in cystic fibrosis. Anesth Analg. 1972;51:413–421.
73 Lamberty JM, Rubin BK. The management of anaesthesia for patients with cystic fibrosis. Anaesthesia. 1985;40:448–459.
74 Walsh TS, Young CH. Anaesthesia and cystic fibrosis. Anaesthesia. 1995;50:614–622.
75 Della RG. Anaesthesia in patients with cystic fibrosis. Curr Opin Anaesthesiol. 2002;15:95–101.
76 Salanitre E, Klonymus D, Rockow H. Anesthetic experience in children with cystic fibrosis of the pancreas. Anesthesiology. 1964;25:801–807.
77 Wainwright CE, Vidmar S, Armstrong DS, et al. Effect of bronchoalveolar lavage-directed therapy on Pseudomonas aeruginosa infection and structural lung injury in children with cystic fibrosis: a randomized trial. JAMA. 2011;306:163–171.
78 Wainwright CE, Grimwood K, Carlin JB, et al. Safety of bronchoalveolar lavage in young children with cystic fibrosis. Pediatr Pulmonol. 2008;43:965–972.
79 Molina-Teran A, Hilliard TN, Saglani S, et al. Safety of endobronchial biopsy in children with cystic fibrosis. Pediatr Pulmonol. 2006;41:1021–1024.
80 Kumle B, Breug R, Boldt J, Munker G. [Anesthesiologic management in the treatment of patients with cystic fibrosis]. Anasthesiol Intensivmed Notfallmed Schmerzther. 2000;35:423–427.
81 Robinson DA, Branthwaite MA. Pleural surgery in patients with cystic fibrosis: a review of anaesthetic management. Anaesthesia. 1984;39:655–659.
82 Price JF. The need to avoid general anaesthesia in cystic fibrosis. J R Soc Med. 1986;79(Suppl 12):10–12.
83 Shelly MP, Lloyd GM, Park GR. A review of the mechanisms and methods of humidification of inspired gases. Intensive Care Med. 1988;14:1–9.
84 Hedley RM, Allt-Graham J. Heat and moisture exchangers and breathing filters. Br J Anaesth. 1994;73:227–236.
85 Chalon J, Loew DA, Malebranche J. Effects of dry anesthetic gases on tracheobronchial ciliated epithelium. Anesthesiology. 1972;37:338–343.
86 Dery R. The evolution of heat and moisture in the respiratory tract during anaesthesia with a non-rebreathing system. Can Anaesth Soc J. 1973;20:296–309.
87 Forbes AR. Humidification and mucus flow in the intubated trachea. Br J Anaesth. 1973;45:874–878.
88 Donaldson SH, Bennett WD, Zeman KL, et al. Mucus clearance and lung function in cystic fibrosis with hypertonic saline. N Engl J Med. 2006;354:241–250.
89 Elkins MR, Robinson M, Rose BR, et al. A controlled trial of long-term inhaled hypertonic saline in patients with cystic fibrosis. N Engl J Med. 2006;354:229–240.
90 Wark P, McDonald VM. Nebulised hypertonic saline for cystic fibrosis. Cochrane Database Syst Rev. 2009. CD001506
91 Tannenbaum E, Prasad SA, Dinwiddie R, Main E. Chest physiotherapy during anesthesia for children with cystic fibrosis: effects on respiratory function. Pediatr Pulmonol. 2007;42:1152–1158.
92 Firth PG, Head CA. Sickle cell disease and anesthesia. Anesthesiology. 2004;101:766–785.
93 Firth PG. Anaesthesia for peculiar cells: a century of sickle cell disease. Br J Anaesth. 2005;95:287–299.
94 Platt OS, Brambilla DJ, Rosse WF, et al. Mortality in sickle cell disease: life expectancy and risk factors for early death [see comments]. N Engl J Med. 1994;330:1639–1644.
95 Castro O, Brambilla DJ, Thorington B, et al. The acute chest syndrome in sickle cell disease: incidence and risk factors. The Cooperative Study of Sickle Cell Disease. Blood. 1994;84:643–649.
96 Vichinsky EP, Styles LA, Colangelo LH, et al. Acute chest syndrome in sickle cell disease: clinical presentation and course. Cooperative Study of Sickle Cell Disease. Blood. 1997;89:1787–1792.
97 Vichinsky EP, Neumayr LD, Earles AN, et al. Causes and outcomes of the acute chest syndrome in sickle cell disease. National Acute Chest Syndrome Study Group. N Engl J Med. 2000;342:1855–1865.
98 Vichinsky EP, Haberkern CM, Neumayr L, et al. A comparison of conservative and aggressive transfusion regimens in the perioperative management of sickle cell disease. The Preoperative Transfusion in Sickle Cell Disease Study Group. N Engl J Med. 1995;333:206–213.
99 Koshy M, Weiner SJ, Miller ST, et al. Surgery and anesthesia in sickle cell disease. Cooperative Study of Sickle Cell Diseases. Blood. 1995;86:3676–3684.
100 Kokoska ER, West KW, Carney DE, et al. Risk factors for acute chest syndrome in children with sickle cell disease undergoing abdominal surgery. J Pediatr Surg. 2004;39:848–850.
101 Vichinsky EP, Neumayr LD, Haberkern C, et al. The perioperative complication rate of orthopedic surgery in sickle cell disease: report of the National Sickle Cell Surgery Study Group. Am J Hematol. 1999;62:129–138.
102 Haberkern CM, Neumayr LD, Orringer EP, et al. Cholecystectomy in sickle cell anemia patients: perioperative outcome of 364 cases from the National Preoperative Transfusion Study. Preoperative Transfusion in Sickle Cell Disease Study Group. Blood. 1997;89:1533–1542.
103 Powars D, Weidman JA, Odom-Maryon T, Niland JC, Johnson C. Sickle cell chronic lung disease: prior morbidity and the risk of pulmonary failure. Medicine (Baltimore). 1988;67:66–76.
104 Boyd JH, Strunk RC, Morgan WJ. The outcomes of sickle cell disease in adulthood are clear, but the origins and progression of sickle cell anemia-induced problems in the heart and lung in childhood are not. J Pediatr. 2006;149:3–4.
105 Koumbourlis AC, Zar HJ, Hurlet-Jensen A, Goldberg MR. Prevalence and reversibility of lower airway obstruction in children with sickle cell disease. J Pediatr. 2001;138:188–192.
106 Gladwin MT, Sachdev V, Jison ML, et al. Pulmonary hypertension as a risk factor for death in patients with sickle cell disease. N Engl J Med. 2004;350:886–895.
107 Miller GJ, Serjeant GR. An assessment of lung volumes and gas transfer in sickle-cell anaemia. Thorax. 1971;26:309–315.
108 Gladwin MT, Vichinsky E. Pulmonary complications of sickle cell disease. N Engl J Med. 2008;359:2254–2265.
109 Firth PG, McMillan KN, Haberkern CM, et al. A survey of perioperative management of sickle cell disease in North America. Paediatr Anaesth. 2011;21:43–49.
110 DeBaun MR, Sarnaik SA, Rodeghier MJ, et al. Associated risk factors for silent cerebral infarcts in sickle cell anemia: low baseline hemoglobin, gender and relative high systolic blood pressure. Blood. 2011;119:3684–3690.
111 Flanagan JM, Frohlich DM, Howard TA, et al. Genetic predictors for stroke in children with sickle cell anemia. Blood. 2011;117:6681–6684.
112 Bishop S, Matheus MG, Abboud MR, et al. Effect of chronic transfusion therapy on progression of neurovascular pathology in pediatric patients with sickle cell anemia. Blood Cells Mol Dis. 2011;47:125–128.
113 Abboud MR, Yim E, Musallam KM, Adams RJ. Discontinuing prophylactic transfusions increases the risk of silent brain infarction in children with sickle cell disease: data from STOP II. Blood. 2011;118:894–898.
114 Adams RJ, Brambilla D. Discontinuing prophylactic transfusions used to prevent stroke in sickle cell disease. N Engl J Med. 2005;353:2769–2778.
115 Ali SB, MooSang M, King L, Knight-Madden J, Reid M. Stroke recurrence in children with sickle cell disease treated with hydroxyurea following first clinical stroke. Am J Hematol. 2011;86:846–850.
116 Crawford MW, Galton S, Naser B. Postoperative morphine consumption in children with sickle-cell disease. Paediatr Anaesth. 2006;16:152–157.
117 Bellet PS, Kalinyak KA, Shukla R, Gelfand MJ, Rucknagel DL. Incentive spirometry to prevent acute pulmonary complications in sickle cell diseases. N Engl J Med. 1995;333:699–703.
118 Tait AR, Knight PR. The effects of general anesthesia on upper respiratory tract infections in children. Anesthesiology. 1987;67:930–935.
119 DeSoto H, Patel RI, Soliman IE, Hannallah RS. Changes in oxygen saturation following general anesthesia in children with upper respiratory infection signs and symptoms undergoing otolaryngological procedures. Anesthesiology. 1988;68:276–279.
120 Levy L, Pandit UA, Randel GI, Lewis IH, Tait AR. Upper respiratory tract infections and general anaesthesia in children. Anaesthesia. 1992;47:678–682.
121 Tait AR, Pandit UA, Voepel-Lewis T, Munro HM, Malviya S. Use of the laryngeal mask airway in children with upper respiratory tract infections: a comparison with endotracheal intubation. Anesth Analg. 1998;86:706–711.