Chapter 12 Embryology and development
After 15 days of development, the human embryo is in the form of a flat, ovoid disc which consists of two layers of cells: the ectoderm dorsally and the endoderm ventrally (Fig. 12.1). The ectoderm is that layer which principally will give rise to the skin and spinal cord. The endoderm forms the alimentary tract.1 At the caudal end of the embryo, the cells of the ectoderm become rounded and heap up, forming an elevation known as the primitive streak.1 Cells from the primitive streak migrate laterally and forwards, insinuating between the ectoderm and endoderm to form a third layer in the embryo called the mesoderm (Figs. 12.1, 12.2). Just in front of the primitive streak, another thickening develops, known as Hensen’s node. From this node, a cord of cells, known as the notochord, migrates forwards between the ectoderm and endoderm (see Fig. 12.2). By about 28 days, the notochord fully demarcates the midline of the embryo1 and induces the formation of the vertebral column around it. Dorsal to the notochord, the ectoderm forms the neural tube, which differentiates into the brain and spinal cord.
On each side of the notochord, the mesoderm of the embryo is thickened to form a longitudinal mass known as the paraxial mesoderm. By the 21st day of development, the paraxial mesoderm starts to be marked by transverse clefts across its dorsal surface. These clefts separate the paraxial mesoderm into segments called somites (Fig. 12.3). The first somites appear in the region of the head, and others appear successively caudally. By about the 30th day of embryonic development, a total of 42–44 somites are formed.1
The clefts demarcating the somites are actually indentations, so the segmentation they create is apparent only along the dorsal aspect of the paraxial mesoderm. Deeply, beneath the surface of the embryo, the paraxial mesoderm remains a single, longitudinally continuous mass.2 Using the transverse clefts as a guide, however, the further development of each somite can be traced.
The 42–44 somites of the human embryo can be named as 4 occipital, 8 cervical, 12 thoracic, 5 lumbar, 5 sacral and 8–10 coccygeal. The first occipital and the last 7–8 coccygeal somites regress and give rise to no permanent structures.1 The remaining three occipital somites are involved in the formation of the occipital region of the skull and the tongue. The other somites form the vertebral column and the trunk.
The cells in the somites are originally epithelial in nature but they gradually change into loosely arranged tissue called mesenchyme (Fig. 12.4). In transverse section, each somite is roughly triangular in outline, presenting ventral and dorsolateral borders, and a medial border facing the neural tube (Fig. 12.5).
Within the somite, two clusters of cells develop. Those cells in the ventral and medial regions of the somite rapidly multiply and form a mass, which, in the past, has been referred to as the sclerotome, but for reasons outlined elsewhere2 the term somitic mesenchyme is used here. These cells are exclusively involved in the formation of the vertebral column. The remaining cells, along the dorsolateral border of the somite, give rise to the musculature and skin of the trunk and are collectively referred to as the dermomyotome.
The fate of the somitic mesenchyme
The notochord lies between the aorta ventrally, and the neural tube dorsally. The neural tube is flanked by the somitic mesenchyme, but the somitic mesenchyme initially does not extend as far medially as the notochord. The notochord is surrounded separately by a continuous column of very loose-meshed mesenchyme called the axial mesenchyme (see Fig. 12.5).2 The density of the axial mesenchyme gradually increases as these cells multiply and surround the notochord (Figs. 12.6, 12.7). Meanwhile, a separate series of events occurs in the somitic mesenchyme.
In the caudal half of each somite, the density of nuclei increases, giving it a darker staining appearance (see Figs 12.4 and 12.7B). The cranial half of the somite remains less dense and is invaded by the developing spinal nerve (see Figs 12.6A and 12.7C). The nerve grows laterally to invade the dermomyotome, and as the nerve increases in length and thickness, the cells of the cranial half of the somite come to be arranged in concentric layers around the nerve.2 In time, the developing nerve occupies most of the entire cranial half of the somite, which itself gives rise to little but perineural tissue. It is the denser, caudal half of each somite that participates in the formation of the vertebral column.
In the caudal half of each somite, two processes develop: a dorsal process and a ventrolateral process.2 The dorsal process spreads dorsally to surround the neural tube and will give rise to the neural arch (see Fig. 12.6B). Hence, it is also referred to as the arcual process. The ventrolateral process extends laterally and gives rise to the costal element of the future vertebrae. Hence, it is also referred to as the costal process (see Fig. 12.6B). In the lumbar region, the costal elements of each vertebra are represented in the form of the transverse processes.
As the axial mesenchyme increases in density, its cells assume a concentric orientation around the notochord. These cells will form the greater part of the future vertebral body, and the portion of the body that they form is referred to as the centrum (see Fig. 12.7). Opposite the lower half of the cranial portion of the adjacent somite, a zone of higher density develops in the axial mesenchyme (see Fig. 12.7). This zone forms the predecessor to the future intervertebral disc.2
While these events take place in the axial mesenchyme, a third process develops in the somitic mesenchyme. This process, known as the ventral or chordal process, extends towards the notochord to blend with the axial mesenchyme just caudal to the zone of the future intervertebral disc.2 In this way, the chordal process connects the somitic mesenchyme with the centrum of the vertebral body, and the vertebral body is eventually formed by the centrum and the terminal portions of the chordal processes from each side.
The dorsal processes of the somitic mesenchyme continue to extend around the sides of the neural tube, and just lateral to the developing dorsal root ganglion, the dorsal processes of adjacent somites blend with one another at the sites of the future zygapophysial joints.2 Elsewhere, the neural arches of adjacent segments are bridged by less dense condensations of mesenchyme that will give rise to the ligaments of the neural arch.
By this stage of development, the shape of the future vertebra is outlined by mesenchymal tissue. Condensations of the axial mesenchyme have surrounded the notochord and have moulded the vertebral body. The future intervertebral disc has condensed in the axial mesenchyme opposite the lower half of the cranial portion of the somitic mesenchyme. The cranial half of each somite has condensed around the developing spinal nerve and will form only perineural tissue. The condensed caudal half of the somitic mesenchyme has formed three processes. A ventral process blends with the axial mesenchyme below the intervertebral disc, while a dorsal process embraces the side of the neural tube. Together, the ventral and dorsal processes outline the future neural arch. The ventrolateral process radiates from the neural arch on each side to outline the future transverse process. At this stage of development, the left and right dorsal processes do not yet meet behind the neural tube and are united only by a membrane.3,4 The neural arch is completed dorsally at a later stage of development.
The fate of the dermomyotome
Initially, two types of cells are evident in the dermomyotome. Epithelial cells cover the dorsolateral surface of the somite and can be recognised as the dermatome. Deep to these lie mesenchymal cells, collectively known as the myotome. Gradually, the cells of the dermatome lose their epithelial character and become incorporated into the myotomal mass, but they remain attached to the overlying ectoderm and give rise to the dermis and subcutaneous tissues.1 The cells of the myotome give rise to muscular tissue.
The myotomal mass maintains its ventrolateral location in relation to the somitic mesenchyme. Opposite the condensed caudal half of the somite it is gradually displaced laterally by the developing ventrolateral process. Opposite the looser cranial half of the somite, it bulges towards the somite but is also indented by the developing spinal nerve (see Fig. 12.7).2
As the spinal nerve divides into a ventral and dorsal ramus at about the 40th day of development,4 the myotome splits into two portions.1 The division occurs along a plane depicted by the developing transverse processes, and the two portions are separated by a septum that forms the future intertransverse ligaments (Fig. 12.8). The dorsal portion of the myotome is known as the epimere, or epaxial portion, and is innervated by the dorsal ramus of the spinal nerve. The ventral portion is known as the hypomere, or hypaxial portion, and is innervated by the ventral ramus of the spinal nerve.
The epimeres throughout the vertebral column divide further into medial and lateral divisions,1,5 which are supplied by the medial and lateral branches of the dorsal rami, respectively. In the lumbar region, the medial division forms the multifidus muscle, while the lateral division forms the iliocostalis and longissimus muscles.
Chondrification
As the mesenchymal models of the vertebrae are being completed, some of the mesenchymal cells change character and become cartilaginous. This occurs at about the 6th week of gestation4 and heralds the onset of the cartilaginous phase of vertebral development.
A pair of chondrification centres appear in the centrum of each vertebra. They rapidly fuse into one centre, which expands to chondrify the entire centrum.1 Chondrification centres also appear in each half of the neural arch. These expand dorsally through the dorsal process of the somitic mesenchyme on each side, and meet one another behind the neural tube to complete the neural arch. From the site of union, a cartilaginous spinous process develops dorsally. The neural arch centres also extend laterally to chondrify the transverse process, and ventrally along the ventral process of the somitic mesenchyme to blend with the chondrifying centrum.
As a consequence of these events, a cartilaginous model of the future vertebra is laid down, but even as chondrification of the vertebral column is being completed, these cartilaginous models start to be replaced by definitive, osseous vertebrae (see Fig. 12.8).
Ossification
Ossification is the third phase of development of the vertebral column. It commences during the 9th–10th weeks of intrauterine life,6 but is not completed until adolescent life. The first process of ossification is called primary ossification and occurs at sites where blood vessels invade the cartilaginous models of the future vertebrae.
The cartilaginous neural arches are invaded from behind to form a primary ossification centre in each half of the neural arch (see Fig. 12.8). The cartilaginous vertebral body is invaded by blood vessels through its anterior and posterior surfaces. Some authorities maintain that these two sets of blood vessels give rise, respectively, to separate ventral and dorsal ossification centres, which rapidly fuse to form a single ossification centre in the middle of the future vertebral body,7 but others maintain that this phenomenon is only a variation that occurs in about 5% of cases.8,9 Another variant is to have two centres lying lateral to one another8 but the most common pattern is to have one single centre.8
The onset of ossification differs according to vertebral level and the part to be ossified. Primary ossification centres in the neural arches first appear at cervicothoracic levels, followed by upper cervical and then thoracolumbar levels. Centres in the neural arches then appear progressively in cranial and caudal directions from these levels.10 Primary centres in the vertebral bodies first appear at lower thoracic and upper lumbar levels, and then progressively appear at levels above and below these.10 In this way, ossification centres are established in the bodies and neural arches of the lumbar vertebrae by the 12th–14th week of gestation.
In the centrum of the vertebral body, the primary ossification centre expands radially and towards the intervertebral discs above and below. It reaches the anterior aspect of the centrum by about 22 weeks of antenatal life, and the posterior aspect by about 25 weeks,11 but ossification does not reach the superior and inferior surfaces of the vertebral body, which remain cartilaginous and form the growth plates of the vertebral body. In the neural arches, ossification extends in all directions from the primary centre: ventrally towards the vertebral body; laterally into the transverse process; and dorsally around the neural tube.
At birth, the lumbar vertebrae are still not completely ossified (Figs. 12.9, 12.10). The bulk of the centrum is ossified, and in lateral radiographs has the appearance of an ovoid block of bone with convex upper and lower surfaces.12–14 Large vascular channels penetrate the anterior and posterior aspects of the centrum,7 and on radiographs of neonatal spines these appear as areas of translucency.14 The upper and lower surfaces of the vertebral body are still covered by the thick cartilage plates, and the combined height of these plates and the intervertebral disc is approximately the same as the height of the ossified lumbar vertebral bodies.12–14 The pedicles and the proximal parts of the laminae and transverse processes are ossified but the spinous processes and the distal parts of the transverse processes are still cartilaginous. The articular processes are ossified for the most part but their distal ends remain cartilaginous.
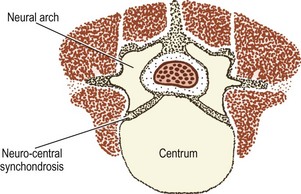
Figure 12.9 A neonatal lumbar vertebra showing the extent of ossification of the centrum and the neural arches.
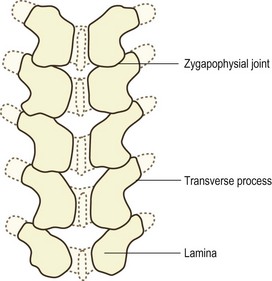
Figure 12.10 A dorsal view of a neonatal lumbar spine showing the extent of ossification of the neural arches.
Ossification continues to spread slowly through the neural arch and its processes. The laminae are fully ossified and unite dorsal to the spinal cord during the first postnatal year.6,15 At this same time the bulk of the spinous process is ossified14 but its dorsal edge remains cartilaginous until puberty, as do the tips of the transverse processes and the ends of the articular processes.
At puberty, secondary ossification centres appear in the cartilaginous tips of the spinous processes, the tips of the transverse processes and in the cartilaginous mamillary processes.6,16 Secondary ossification centres may appear in the tips of the inferior articular processes but this phenomenon does not occur regularly; it is described further in the section on the zygapophysial joints.
The secondary ossification centres of each lumbar vertebra are separated from the rest of the vertebra by a narrow interval of cartilage and remain separated during the final periods of spinal growth. Gradually, this intervening cartilage is replaced by bone, and the secondary centres fuse with the rest of the vertebra by about the 25th year of life.6
The fate of the notochord
As chondrification of the vertebral bodies proceeds, the cells of the notochord appear to be squeezed out of the vertebral body into the intervertebral discs (see Fig. 12.7)7,17,18 and the notochord is progressively narrowed until it forms little more than a streak of tissue on the vertebral body, known as the mucoid streak. Expansion of the ossification centre of the vertebral centrum destroys the mucoid streak, and in general, any vestige of the notochord in the vertebral body is obliterated.7
In about 7% of cases, ossification does not completely obliterate the region of the notochord, and a vertical canal may persist in the vertebral body.19 These canals are most frequently filled with fibrocartilage or fibrous tissue, but rarely, pockets of notochordal cells may persist in parts of the canal.19
Development of the intervertebral disc
In the primitive mesenchymal intervertebral disc, the cells gradually come to be arranged in concentric layers, lying in parallel rows between one vertebra and the next.20 This arrangement foreshadows the future concentric structure of the lamellae of the anulus fibrosus (Fig. 12.11A).
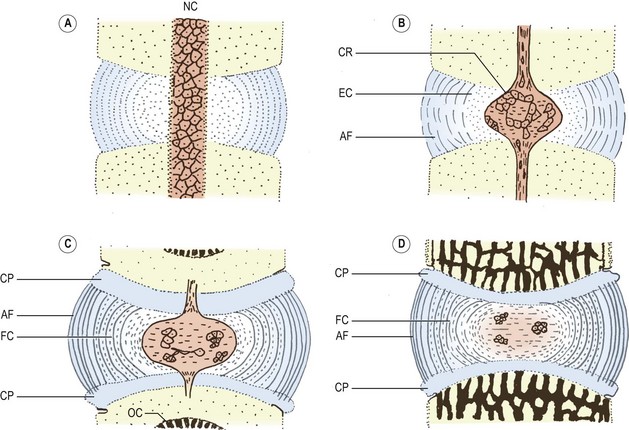
Figure 12.11 Stages in the development of the intervertebral disc. (Based on Peacock 1951.20) (A) A mesenchymal disc in which the central cells surround the notochord (NC), and the peripheral cells are arranged in a radial pattern indicative of the future lamellae of the anulus fibrosus. (B) The notochord has expanded and its cells form the chorda reticulum (CR). Mesenchymal cells surrounding the notochord have transformed into embryonic cartilage (EC), and the peripheral cells have formed the orientated collagen fibres of the anulus fibrosus (AF). (C) The disc consists of an expanded notochord with fewer cells, surrounded by fibrocartilage (FC) and the collagenous anulus fibrosus which attaches to the cartilaginous plates of the vertebrae (CP). Ossification centres (OC) are present in the centra. (D) A neonatal disc.
Towards the centre of the disc, the cells are irregularly arranged around the notochord, and gradually the cells closer to the notochord take on the appearance of embryonic cartilage (Fig. 12.11B).20 At about 55 days of development, the notochord expands in the centre of the disc, its cells being separated into strands and groups, called the chorda reticulum, embedded in an amorphous mucoid substance (Fig. 12.11B). The expanded notochord is surrounded by embryonic cartilage, and around the perimeter of the disc, collagen fibres appear to form the anulus fibrosus.
Collagen fibres are deposited in the anulus fibrosus as early as the 10th week of gestation,21 and their orientation is the same as that in the adult.22 Their ends are inserted into the cartilage plates that cover the superior and inferior aspects of the vertebral bodies. Fibres in the anulus fibrosus are quite evident in the 4th month and are well developed by 5–6 months.7 Accompanying the development of the anulus fibrosus, the anterior and posterior longitudinal ligaments condense out of the perivertebral mesenchyme during the 7th–9th weeks.7
In the centre of the intervertebral disc, the notochord continues to expand radially, and the perichordal cartilage assumes a looser arrangement.20 The cartilage cells closer to the anulus fibrosus undergo a transition to fibrocartilage, whose collagen fibres are arranged in parallel sheets like the fibres of the anulus fibrosus (Fig. 12.11C).
At birth, the notochordal area is formed essentially by an amorphous mucoid material that contains only a few small groups of notochordal cells. The notochordal area is surrounded by a capsule of fibrocartilage, and beyond this lies the collagenous anulus fibrosus. At this stage, the structure of the anulus resembles that seen in the adult (Fig. 12.11D).
After birth, some of the notochordal cells may persist in the disc, but eventually all notochordal cells undergo necrosis during infancy.23,24 After the age of 4 years, no viable notochordal cells remain and the centre of the disc contains only the notochordal mucoid material and the perichordal fibrocartilage.
In the neonate and infant, the nucleus pulposus is wedge shaped in the median section, with its main mass located posteriorly in the disc.11 By 2 years of age this shape is reversed, and the main mass lies anteriorly.11 From the 4th to 8th years of life, the nucleus assumes an elliptical shape and occupies the centre of the disc. This final change in position occurs as the child masters upright weight-bearing and gait, and accompanies the development of the lumbar lordosis and a rapid increase in height of the lumbar vertebrae and discs.11
Between the ages of 2 and 7 years, the lumbar discs change their shape from a biconcave disc bounded by convex bony surfaces to a biconvex disc bounded by concave surfaces,11 and throughout childhood the lumbar discs undergo a major increase in height. The L4–5 disc, for example, increases from 3 mm in height to about 10 mm, between birth and the age of 12.
Growth of the vertebral bodies
The neurocentral joints persist into childhood but gradually the cartilage is ossified and the pedicles fuse with the centrum by about the age of 6 years.14 In fusing with the centrum, the pedicles contribute to the formation of the vertebral body, which is therefore formed largely by the ossified centrum but also by the ventral ends of the neural arches.
Horizontal growth
Horizontal growth of the vertebral body occurs by periosteal ossification,12,13,25 and from birth to the age of 7 years the anteroposterior diameter of a typical lumbar vertebral body increases from 3 mm to about 22 mm12 or 27 mm.11 During the same period, the lateral diameter increases from 7 mm to about 36 mm.11 By the age of 17 years, the anteroposterior diameter reaches 34 mm.12 Between the ages of 5 and 13 years, the transverse diameter of the lumbar vertebral bodies in males increases by about 26% at the L1 and L3 levels, and by 30% at the L5 level. In females, the corresponding increases are about 15% and 22%.26 From puberty to adulthood the transverse diameters increase by 5–10% in both males and females. The mean values for the L1, L3 and L5 vertebrae increase from 38, 42 and 48 mm to 42, 44 and 52 mm, respectively.26
Longitudinal growth
Longitudinal growth of the vertebral bodies occurs as a result of the proliferation and ossification of the cartilages remaining on the superior and inferior surfaces of the vertebral body.13,27 These cartilages cover the entire superior and inferior surfaces, but also overlap onto the anterior, lateral and posterior margins of the vertebral body (Fig. 12.12).7,14,28 On their discal aspect these cartilages blend with the developing intervertebral disc. They are directly confluent with the fibrocartilage of the developing nucleus, and they anchor the fibres of the anulus fibrosus (see Fig. 12.12). On the vertebral aspect of each plate, the cartilage cells are arranged in vertical columns,17,27 and ossification occurs by the same process seen in the metaphyses of long bones.29
The cells furthest away from the cartilage plate are surrounded by calcified matrix and undergo ossification, whereupon they are incorporated into the vertebral body. Longitudinal growth occurs as these cells are replaced by division of cells closer to the main body of the cartilage plate. Growth continues as long as this replacement continues, and the rate of growth appears to be equal at both the upper and lower growth plates.25,30
Between birth and the age of 5 years, a typical lumbar vertebra increases in height from 5 mm to about 15 mm11,26 or 18 mm.12 From the age of 5 to the age of 13, it increases to about 22 mm, and reaches 25 mm by adulthood.26 Other studies estimate the sizes of the vertebrae at the age of 13 and at adulthood to be 26 mm and 34 mm, respectively.12 The average vertical dimensions of all the lumbar vertebrae and intervertebral discs at various ages are shown in Table 12.1. The dramatic increase in size during childhood is readily apparent. During adolescence, females exhibit somewhat smaller average dimensions than males, but approach male dimensions more closely by adulthood.
The extent of longitudinal growth of the central region of the vertebral body appears to be genetically determined, but the longitudinal growth of the peripheral portions is dependent on activity associated with weight-bearing in the erect posture.11 With assumption of the lumbar lordosis, the nucleus pulposus comes to be located in the centre of each intervertebral disc,11 and this location of the nucleus acts as a stimulus for growth of the more peripheral parts of the vertebral body.11 It is as if the peripheral parts grow to attempt to surround the nucleus, and this differential growth accounts for the relatively concave shape of the superior and inferior surfaces of the developing vertebral bodies.
Longitudinal growth of the vertebral bodies continues throughout childhood and adolescence, but gradually the rate of growth slows down and is completed between the ages of 18 and 25.16 As ossification ceases, the growth plates become thinner, and the vertebral surface of the growth plate is sealed off from the vertebral body by both a calcified layer of cartilage and the development of the subchondral bone plate at each end of the vertebral body. The hyaline and fibrocartilage remaining on the surfaces of the body then becomes the vertebral endplate of the intervertebral disc.
During vertebral growth the cartilaginous growth plates are nourished by blood vessels that ascend and descend along the outer surfaces of the vertebral body and enter the peripheral edges of the growth plates. They then run within the growth plate towards its centre, raising ridges in the cartilage over the upper and lower surfaces of the vertebral body. These ridges radiate from the centre of the growth plate to its perimeter and are more marked anteriorly. As growth slows down, the vessels in these ridges are gradually obliterated, and the ridges disappear.28
Ring apophysis
During the growth period, a separate series of events involve the perimeter of the cartilaginous growth plates but do not contribute to growth. These events relate to the formation of the ring apophyses of the vertebrae (see Ch. 1). In the edges of the cartilaginous plates, where they overlap the anterior, lateral and posterior margins of the vertebral body, foci of calcification appear, at the ages of 6–8 years in girls and 7–9 years in boys.14 These foci are subsequently ossified as a result of vascular infiltration. At first, many such foci surround the upper and lower margins of the vertebral body, but by about the age of 12 years they coalesce to form a single rim, or a ring. This ring surrounds the entire perimeter of the vertebral body but is better developed anteriorly and laterally. It remains separated from the rest of the vertebral body by a thin layer of hyaline cartilage but eventually fuses with the vertebra, some time between the ages of 14 and 1514 or 16 and 21 (see Fig. 12.12).31,32
At no time does the ring apophysis contribute to growth, but its fusion with the rest of the vertebral body signals the cessation of longitudinal growth. One effect of the ring apophysis is that, because it develops as a result of ossification of the margins of the cartilage growth plate, it incorporates those fibres of the anulus fibrosus that insert into the perimeter of the plate (Fig. 12.12D). This explains why the peripheral fibres of the adult anulus have a bony attachment, while the more central fibres are inserted into the vertebral endplate.
Development of the zygapophysial joints
Compared to the embryology and development of the vertebral bodies, the development of the lumbar zygapophysial joints has received scant attention. There are few descriptions in the English language literature, although some major studies have been published in the continental literature.33–36 Notwithstanding this relative neglect, there are some fascinating and clinically relevant aspects of the development of these joints.
The lumbar zygapophysial joints develop from the mesenchyme of the neural arches, rudimentary mesenchymal articular processes appear at about 32 days of development,3 and the mesenchymal processes of consecutive vertebrae eventually meet one another at about 50 days.34 The future joint space is initially surrounded and filled with mesenchyme but as the articular processes chondrify, this tissue gradually recedes to form the articular capsule, any intra-articular structures, and a joint space. Chondrification commences at about 50 days,3 and ossification by about 100 days.
Although definitive joints are formed by the 9th month of gestation,34,36 at birth the articular processes are incompletely ossified. They are flat and spatula-like, and their tips are still covered by cartilage.15 The superior articular process is rudimentary and is about half the length of the inferior articular process, but undergoes extensive development during the first 2 years of life.
At birth, the lumbar zygapophysial joints are all orientated in a coronal plane, like the joints of the thoracic vertebrae, but during postnatal growth their orientation changes to that seen in adults by about the age of 11 years (Fig. 12.13).34,36–38 Rotation is achieved by differential rates and extents of ossification of the articular processes.39
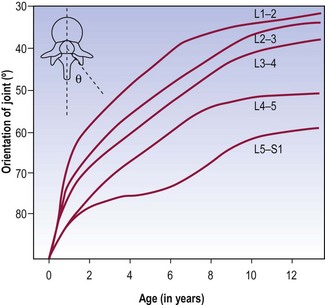
Figure 12.13 The orientation of the lumbar zygapophysial joints as a function of age during growth.
(Based on Lutz 1967.34)
At birth, bone occurs in the medial and basal parts of both inferior and superior articular processes. Further ossification occurs in three directions: towards the apex of each articular process along the medial margins of the joint; towards the joint surface leaving a joint cartilage; and around the lateral aspects of each articular process (Fig. 12.14).39 Medial growth occurs rapidly but ceases at the age of 6 months. After this age the medial margin of the joint is resorbed and remodelled as the neural arch expands to assume adult proportions.39,40 Lateral ossification is more protracted as further cartilage is laid down as ossification proceeds. With medial ossification completed, continued lateral ossification brings about the apparent rotation of the joint (Fig. 12.15). The joints are fully ossified by about 7–9 years of age, by which time the adult orientation is virtually fully established (see Fig. 12.13).
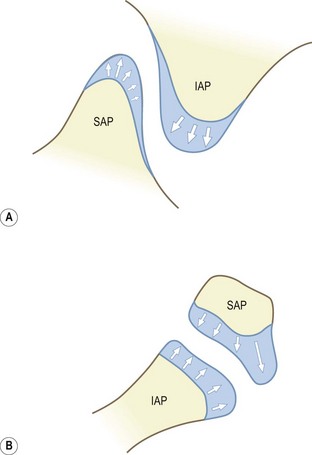
Figure 12.14 The directions of ossification of the articular processes. (A) Lateral view. (B) Top view. (Based on Reichmann 1971.39) IAP, inferior articular process; SAP, superior articular process. In the neonate only the basal regions of each articular process are ossified. Their tips are covered by cartilage into which ossification extends.
Variations in the extent of developmental rotation account for the variations in orientation of the lumbar zygapophysial joints seen in adults (see Ch. 3). Joints with a more pronounced posterior, lateral growth would exhibit curvatures (see Fig. 12.15A); those with a more pronounced lateral growth and less dorsal growth would tend to remain planar (see Fig. 12.15B).
The cause of joint ‘rotation’ is unknown. It may be a genetically determined property of the lumbar zygapophysial joints but other explanations have been suggested. Because it occurs as the child learns to stand erect and begins to use the multifidus muscle in everyday activities, some authors38 attribute rotation of the lumbar zygapophysial joints to the action of multifidus (see Ch. 9). By pulling on the mamillary processes, the multifidus swings the lateral extremity of the superior articular process to a more dorsal position, thereby rotating the plane of the joint or imparting a curvature to it.
The articular processes continue to grow until about the age of 20;41 infrequently, secondary ossification centres (epiphyses) may appear in the tip of the inferior articular processes at puberty. The exact incidence of these centres is unknown but, when formed, they fuse with the main part of the inferior articular process between 15 and 21 years of age.14,42,43
Developmental anomalies
The developmental anomalies of the lumbar spine are vast and varied. In general they are thoroughly described in major textbooks of spinal morphology and radiology,14,15,44,45 and in research papers specifically addressing this issue.46–50
Systematically, lumbar vertebral anomalies can be classified into:
Part or all of a vertebral body may fail to be formed. When this occurs, the body assumes a wedge shape, with the orientation of the wedge dependent on which part of the body fails: failure of the anterior half of the body leads to anterior wedging; failure of the posterior part causes posterior wedging; and lateral failure leads to lateral wedging. The embryological basis for these deformities is described in detail elsewhere.14
Individual components of the neural arches may fail to develop. In particular, a pedicle may be absent or not ossified,51–53 and articular processes, mostly inferior articular processes, may fail to develop.54–57
The most common form of non-union is spina bifida, in which the laminae of a vertebra, most commonly L5, fail to unite dorsally behind the cauda equina. This may simply be a failure of ossification of an otherwise united cartilaginous neural arch, but spina bifida can be associated with minor and quite major abnormalities of the dural sac, cauda equina and spinal cord.14,44,46
The secondary ossification centres of the inferior articular processes may fail to unite with the articular process, or may be late in so doing. Under these circumstances, the isolated ossicle formed by the secondary centre may mimic a fractured articular process.58,59 United epiphyses occur in asymptomatic58–62 and symptomatic58,59,61–64 individuals, and it is not clear under what circumstances they can cause symptoms. It seems possible that if they were detached from the articular process, they could interfere with the mechanics of the zygapophysial joint like a loose body;15 compression of the underlying spinal nerve roots by a dislocated epiphysis has been described.65 Estimates of the incidence of united epiphyses vary from 1.5%60,65 to 14%.66
Alterations in vertebral number and identity affect principally the lumbosacral region, where the last lumbar vertebra may become incorporated into the sacrum (sacralisation), thereby reducing the number of lumbar vertebrae to four; or conversely the first sacral vertebra may be mobile (lumbarisation), in which case the number of lumbar vertebrae increases to six. Various intermediate states of these same processes occur, with vertebrae showing features of partial lumbarisation or sacralisation.14,46,48,50 None of these anomalies, per se, are the cause of symptoms,67 unless some other disease process or injury is superimposed.
However, anomalous vertebrae may form joints – with the sacrum below or with the ilium laterally – and these joints may be injured or strained, and become symptomatic. The diagnosis is made by anaesthetising the putatively painful joint.68
Articular tropism
One of the consequences of the rotation that the lumbar zygapophysial joints undergo is that the extent of rotation of the left and right joints at any segmental level may not be equal. Thus, the joints may be asymmetrically orientated, a condition referred to as articular tropism. The incidence of tropism is about 20% at all lumbar levels,48,66 but may be as high as 30% at the lumbosacral level,46 with 20% of lumbosacral zygapophysial joints showing an asymmetry greater than 10°.69
Early views suggested that articular tropism predisposed to the development of osteoarthritis in the more asymmetrical joint and to consequent narrowing of the related intervertebral foramen.70 Others felt that asymmetry allowed unequal rotation of the intervertebral joint70 and rendered it more susceptible to ‘ligamentous injury’ (although exactly which ligaments were likely to be injured was not specified).49,69 Modern interest has focused on the significance of articular tropism in torsion injuries of the intervertebral disc, disc degeneration and disc herniation.
Biomechanical studies have shown that asymmetrical zygapophysial joints do not equally resist posteroanterior shear stresses applied to the intervertebral joint. The unequal load-sharing causes the intervertebral joint to rotate whenever it is subjected to shear stress, as in weight-bearing or flexion (see Ch. 8). The upper vertebra in the joint rotates towards the side of the more coronally orientated joint.71 Consequently, the anulus fibrosus is subjected to inordinate stresses during weight-bearing and flexion movements of the lumbar spine. Repeated insults sustained in this way could damage the anulus fibrosus.
Post-mortem studies support this contention. Radial fissures in the anulus fibrosus are a sign of injury to the intervertebral disc (see Ch. 15), and post-mortem studies reveal that over 80% of unilateral fissures occur in intervertebral joints whose zygapophysial joints are asymmetrical by more than 10°. In 80% of these cases the fissure points towards the side of the more obliquely (coronally) orientated joint.72 However, clinically, studies differ.
In one study, based on plain radiographs, articular tropism was found to occur in as many as 90% of patients presenting with low back pain and sciatica, with the symptoms occurring on the side of the more obliquely set joint.73 Later studies, however, using CT scans, found no relationship between articular tropism and either the presence or side of protrusion.74,75 On the other hand, another study did find a statistically significant relationship, not with disc herniation but between tropism seen on CT scans and disc degeneration seen on magnetic resonance imaging.76 In that study tropism was defined as joint asymmetry greater than 5°.
An exhaustive study, based on CT discography, established the prevalence of tropism in 108 patients with back pain.77 Joint asymmetry was normally distributed in that population, with a mean of 0°, a one standard deviation range of 7° and a two standard deviation range of 15°. No significant association was found between tropism and either disc degeneration or discogenic pain.
Thus, despite earlier enthusiasm, and despite the purported biomechanical significance of tropism, there is no clinically useful relationship between tropism and disc degeneration, disc herniation or discogenic pain. Whether or not tropism has a relationship to torsion injuries of the disc (see Ch. 15) has yet to be explored.
1 Hamilton WJ, Boyd JD, Mossman HW. Human Embryology, 3rd ed. Cambridge: Heffer; 1962.
2 Verbout AJ. The development of the vertebral column. Adv Anat Embryol Cell Biol. 1985;90:1-122.
3 O’Rahilly R, Meyer DB. The timing and sequence of events in the development of the human vertebral column during the embryonic period proper. Anat Embryol. 1979;157:167-176.
4 O’Rahilly R, Muller F, Meyer DB. The human vertebral column at the end of the embryonic period proper. J Anat. 1980;131:565-575.
5 Winckler G. Les muscles profonds du dos chez l’homme. Arch Anat Histol Embryol. 1948;31:1-58.
6 Williams PL, editor. Gray’s Anatomy, 38th ed, Edinburgh: Churchill Livingstone, 1995.
7 Ehrenhaft JC. Development of the vertebral column as related to certain congenital and pathological changes. Surg Gynecol Obst. 1943;76:282-292.
8 Bagnall KM, Harris PF, Jones PRM. A radiographic study of variations of the human fetal spine. Anat Rec. 1984;208:265-270.
9 Cohen J, Currarino G, Neuhauser EBD. A significant variant in the ossification centers of the vertebral bodies. Am J Roentgenol. 1956;76:469-475.
10 Bagnall KM, Harris PF, Jones PRM. A radiographic study of the human fetal spine. 2. The sequence of development of ossification centres in the vertebral column. J Anat. 1997;124:791-802.
11 Taylor JR. Growth of the human intervertebral discs and vertebral bodies. J Anat. 1975;120:49-68.
12 Brandner MF. Normal values of the vertebral body and intervertebral disc index during growth. Am J Roentgenol. 1970;110:618-627.
13 Caffey J. Pediatric X-ray Diagnosis, 6th ed. Chicago: Year Book Medical Publishers; 1972.
14 Schmorl G, Junghanns H. The Human Spine in Health and Disease, 2nd American ed. New York: Grune & Stratton; 1971. 18
15 Hadley LA. Anatomico-roentgenographic Studies of the Spine. Springfield: Thomas; 1964.
16 Carpenter EB. Normal and abnormal growth of the spine. Clin Orthop. 1961;21:49-55.
17 Coventry MB, Ghormley RK, Kernohan JW. The intervertebral disc: its microscopic anatomy and pathology. Part I. Anatomy, development and physiology. J Bone Joint Surg. 1945;27:105-112.
18 Keyes DC, Compere EL. The normal and pathological physiology of nucleus pulposus of the intervertebral disc. J Bone Joint Surg. 1932;14:897-938.
19 Taylor JR. Persistence of the notochordal canal in vertebrae. J Anat. 1972;111:211-217.
20 Peacock A. Observations on the pre-natal development of the intervertebral disc in man. J Anat. 1951;85:260-274.
21 Hickey DS, Hukins DWL. Collagen fibril diameters and elastic fibres in the annulus fibrosus of human fetal intervertebral disc. J Anat. 1981;133:351-357.
22 Hickey DS, Hukins DWL. X-ray diffraction studies of the arrangement of collagen fibres in human fetal intervertebral disc. J Anat. 1980;131:81-90.
23 Coventry MB. Anatomy of the intervertebral disk. Clin Orthop. 1969;67:9-15.
24 Meachim G, Cornah MS. Fine structure of juvenile human nucleus pulposus. J Anat. 1970;107:337-350.
25 Knuttson F. Growth and differentiation of the post-natal vertebrae. Acta Radiol. 1961;55:401-408.
26 Taylor JR, Twomey LT. Sexual dimorphism in human vertebral body shape. J Anat. 1984;138:281-286.
27 Bick EM, Copel JW. Longitudinal growth of the human vertebrae. J Bone Joint Surg. 1950;32A:803-814.
28 Donisch EW, Trapp W. The cartilage endplate of the human vertebral column (some considerations of postnatal development). Anat Rec. 1971;169:705-716.
29 Ham AW, Cormack DH. Histology, 8th ed. Philadelphia: Lippincott; 1979. 373
30 Gooding CA, Neuhauser EBD. Growth and development of the vertebral body in the presence and absence of normal stress. Am J Roentgenol. 1965;93:388-394.
31 Calvo LJ. Observations on the growth of the female adolescent spine and its relation to scoliosis. Clin Orthop. 1957;10:40-46.
32 Walmsley R. Growth and development of the intervertebral disc. Edin Med J. 1953;60:341-364.
33 Guntz E. Die Erkrankungen der Zwischenwirbelgelenke. Arch Orthop Unfallchir. 1933–1934;34:333-355.
34 Lutz G. Die Entwicklung der kleinen Wirbelgelenke. Z Orth. 1967;104:19-28.
35 Tondury G. Beitrag zur Kenntnis der klein Wirbelgelenke. Z Ant Entwickl Gesch. 1940;110:568-575.
36 Tondury G. Anatomie fonctionelle des petits articulations du rachis. Ann Med Phys. 1972;15:173-191.
37 Huson A. Les articulations intervertébrales chez le foetus humain. Comptes Rendus de l’Association des Anatomistes. 1967;52:676-683.
38 Odgers PNB. The lumbar and lumbosacral diarthrodial joints. J Anat. 1933;67:301-317.
39 Reichmann S. The postnatal development of form and orientation of the lumbar intervertebral joint surfaces. Z Anat Entwickl-Gesch. 1971;133:102-121.
40 Reichmann S, Lewin T. Growth processes in the neural arch. Z Anat Entwickl-Gesch. 1971;133:89-101.
41 Lewin T. Osteoarthritis in lumbar synovial joints. Acta Orthop Scand. 1964;73(Suppl.):1-112.
42 Hadley LA. Secondary ossification centers and the intraarticular ossicle. Am J Roentgenol. 1956;76:1095-1101.
43 McMurrich JP. The Development of the Human Body, 6th ed. Philadelphia: Blakiston; 1919. 167
44 Epstein BS. The Spine. A Radiological Text and Atlas, 4th ed. Philadelphia: Lea & Febiger; 1976.
45 McCulloch JA. Congenital anomalies of the lumbosacral spine. In: Genant HK, editor. Spine Update 1984. San Francisco: Radiology Research and Education Foundation; 1983:43-49. Ch. 6
46 Brailsford JF. Deformities of the lumbosacral region of the spine. Br J Surg. 1929;16:562-627.
47 Pheasant HC, Swenson PC. The lumbosacral region. A correlation of the roentgenographic and anatomical observations. J Bone Joint Surg. 1942;24:299-306.
48 Southworth JD, Bersack SR. Anomalies of the lumbosacral vertebrae in five hundred and fifty individuals without symptoms referable to the low back. Am J Roentgenol. 1950;64:624-634.
49 Willis TA. An analysis of vertebral anomalies. Am J Surg. 1929;6:163-168.
50 Willis TA. Anatomical variations and roentgenographic appearance of the low back in relation to sciatic pain. J Bone Joint Surg. 1941;23:410-416.
51 De Boeck M, De Smedy E, Potvliege R. Computed tomography in the evaluation of a congenital absent lumbar pedicle. Skeletal Radiol. 1982;8:197-199.
52 Macleod S, Hendry GMA. Congenital absence of a lumbar pedicle. Pediatr Radiol. 1982;12:207-210.
53 Yousefzadeh DK, El-Khoury GY, Lupetin AR. Congenital aplastic–hypoplastic lumbar pedicle in infants and young children. Skeletal Radiol. 1982;7:259-265.
54 Arcamano JP, Karas S. Congenital absence of the lumbosacral articular process. Skeletal Radiol. 1982;8:133-134.
55 Keim HA, Keagy RD. Congenital absence of lumbar articular facets. J Bone Joint Surg. 1967;49A:523-526.
56 Klinghoffer LK, Muedock MM, Hermal MB. Congenital absence of lumbar articular facets. Clin Orthop. 1975;106:151-154.
57 Phillips MR, Keagy RD. Congenital absence of lumbar articular facets with computerized axial tomography documentation. Spine. 1988;13:676-678.
58 Bailey W. Anomalies and fractures of the vertebral articular processes. JAMA. 1937;108:266-270.
59 Rendich RA, Westing SW. Accessory articular process of the lumbar vertebrae and its differentiation from fracture. Am J Roentgenol. 1933;29:156-160.
60 Farmer HL. Accessory articular processes in the lumbar spine. Am J Roentgenol. 1936;36:763-767.
61 Nichols BH, Shiflet EL. Ununited anomalous epiphyses of the inferior articular processes of the lumbar vertebrae. J Bone Joint Surg. 1933;15:591-600.
62 Fulton WS, Kalbfleisch WK. Accessory articular processes of the lumbar vertebrae. Arch Surg. 1934;29:42-48.
63 King AB. Back pain due to loose facets of the lower lumbar vertebrae. Bull Johns Hopkins Hosp. 1955;97:271-283.
64 Pellegrini VD, Hardy JH. The absent lumbosacral articular process. A report of three cases and review of the literature. Clin Orthop. 1983;175:197-201.
65 Pou-Serradell A, Casademont M. Syndrome de la queue de cheval et présence d’appendices apophysaires vertébraux, ou apophyses articulaires accessoires, dans la région lombaire. Rev Neurol. 1972;126:435-440.
66 Horwitz T, Smith RM. An anatomical, pathological and roentgenological study of the intervertebral joints of the lumbar spine and of the sacroiliac joints. Am J Roentgenol. 1940;43:173-186.
67 Splithoff CA. Lumbosacral junction: roentgenographic comparisons of patients with and without backache. JAMA. 1953;152:199-201.
68 Jonsson B, Stromqvist B, Egund N. Anomalous lumbosacral articulations and low-back pain: evaluation and treatment. Spine. 1989;14:831-834.
69 Badgley CE. The articular facets in relation to low-back pain and sciatic radiation. J Bone Joint Surg. 1941;23:481-496.
70 Von Lackum HL. The lumbosacral region. An anatomic study and some clinical observations. JAMA. 1924;82:1109-1114.
71 Cyron BM, Hutton WC. Articular tropism and stability of the lumbar spine. Spine. 1980;5:168-172.
72 Farfan HF, Huberdeau RM, Dubow HI. Lumbar intervertebral disc degeneration. The influence of geometrical features on the pattern of disc degeneration – a post-mortem study. J Bone Joint Surg. 1972;54A:492-510.
73 Farfan HF, Sullivan JD. The relation of facet orientation to intervertebral disc failure. Can J Surg. 1967;10:179-185.
74 Cassidy JD, Loback D, Yong-Hing K, et al. Lumbar facet joint asymmetry: intervertebral disc herniation. Spine. 1992;17:570-574.
75 Hagg O, Wallner A. Facet joint asymmetry and protrusion of the intervertebral disc. Spine. 1990;15:356-359.
76 Noren R, Trafimow J, Andersson GBJ, et al. The role of facet joint tropism and facet angle in disc degeneration. Spine. 1991;16:530-532.
77 Vanharanta H, Floyd T, Ohnmeiss DD, et al. The relationship of facet tropism to degenerative disc disease. Spine. 1993;18:1000-1005.