7 Electrical events
Structure of the Plasma Membrane
In common with cells elsewhere, the plasma membrane of neurons is a double layer (bilayer) of phospholipids made up of phosphate heads facing the aqueous media of the extracellular and intracellular spaces, and paired lipid tails forming a fatty membrane in between (Figure 7.1). The phosphate layer is water-soluble (hydrophilic, or polar) and the double lipid layer is water-insoluble (hydrophobic, or non-polar).
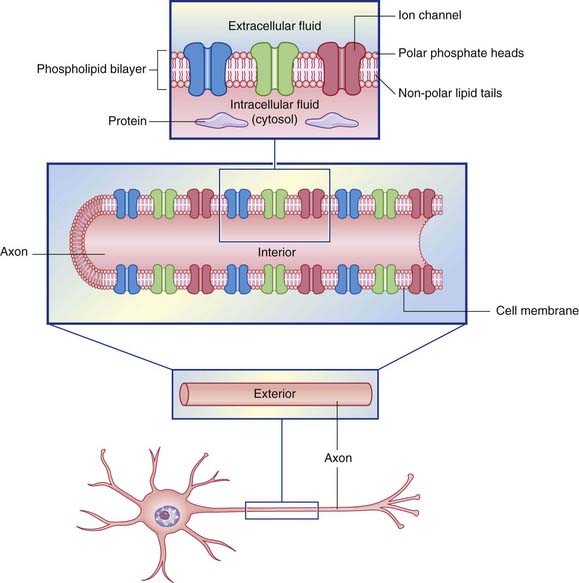
Figure 7.1 Structure of the neuronal cell membrane. The only membrane proteins shown here are ion channels.
Ion channels
Several channel categories are recognized, of which the first three are of immediate relevance.
Figure 7.2 depicts the three passive channels concerned with generating the resting potential.
The resting membrane potential
The membrane potential of the resting (inactive) neuron is generated primarily by differences in concentration of the sodium (Na+) and potassium (K+) ions dissolved in the aqueous environments of extracellular fluid (ECF) and cytosol. In Table 7.1, it can be seen that potassium is 20 times more concentrated in the cytosol; sodium is 10 and chloride 3.8 times more concentrated in the ECF.
In Figure 7.3, a voltmeter is connected to electrodes inserted into the ECF surrounding an axon. One of the electrodes has been inserted into a glass pipette having a minute tip. On the left side of the figure, both electrode tips are in the ECF, and there is no voltage difference; a zero value is recorded. On the right side, the pipette has been lowered, puncturing the plasma membrane of the axon and admitting the intracellular fluid of the cytosol. The electrical charge now reveals a potential (voltage) difference of −70 mV. In practice, the membrane potential ranges from −60 mV to −80 mV in different neurons. These values represent the resting membrane potential, i.e. when impulses are not being conducted.
Resting membrane permeability
Potassium ions
Converting the natural log to log10 and resolving the numeric fractions yields
Repeating the exercise for sodium and chloride yields
The value of the resting potential can be calculated using the Goldman equation, from the relative distributions of the three principal ions involved (Table 7.1), and their membrane permeabilities.
Sodium pump
The resting potential needs to be stabilized, because of the tendency of Na+ ions to leak inward and K+ to leak outward along their concentration gradients. Stability is assured by the Na+–K+ pump making appropriate corrections for their passive flows. This channel is capable of simultaneously extruding N+ and importing K+. Three sodium ions are exported for every two potassium ions imported (Figure 7.4). In both cases, the movement is against the existing concentration gradient. The required energy for this activity is provided by the ATPase enzyme that converts ATP to ADP. The greater the amount of Na+ in the cytosol, the greater is the activity of the enzyme.
As mentioned in Chapter 6, the axonal degeneration occurring in multiple sclerosis is attributable to failure of the sodium pump along the denuded axolemma. This leads to Na+ overload, which in turn promotes excess Ca2+ release from intracellular stores.
Response to Stimulation: Action Potentials
Electrotonic potentials
The initial target cell response to excitatory stimulation takes the form of local, graded or electrotonic potentials (ETPs). Positive ETPs on multipolar neurons are usually on one or more dendrites in receipt of excitatory synapses. At a low frequency of stimulation, small, decremental waves of depolarization extend for 50–100 µm along the affected dendrites, dying away after 2 or 3 ms (Figure 7.5). With increasing frequency, the waves undergo stepwise temporal summation to form progressively larger waves continuing on over the surface of the soma. Spatial summation occurs when waves traveling along two or more dendrites coalesce on the soma (Figure 7.6). About 15 mV of depolarization, to a value of −55 mV, brings the neuron to threshold (firing level) at its most sensitive region, or trigger point, in the initial segment of the axon (Figure 7.7). The initial segment is the first region to ‘give way’ at threshold voltage, because it is exceptionally rich in voltage-gated sodium channels. When the level of depolarization (the generator potential) reaches threshold, nerve impulses in the form of action potentials are suddenly fired off.
The shape of action potentials
A single action potential is depicted in Figure 7.8. The spike segment of the potential commences when the local response reaches threshold value at −55 mV. The rising phase of depolarization passes beyond zero to include an overshoot phase reaching about +35 mV. Overshoot phase includes the rising and falling phases above zero potential. The falling phase planes out in a brief after-depolarization, prior to an undershoot phase of after-hyperpolarization where the membrane potential reaches about −75 mV before returning to baseline.
It should be pointed out that standard representations such as this figure show the voltage changes plotted against a time base. When direction is substituted for time, it becomes obvious that the time-based picture matches the sequence in a peripheral sensory neuron. For all multipolar neurons, the representation should be the reverse (Figure 7.7).
When the local response to stimulation has depolarized the membrane to threshold, the sudden increase in depolarization is brought about by the opening of voltage-gated sodium channels (Figure 7.9). Sodium entry produces further depolarization, and the positive feedback causes the remaining Na+ channels of the trigger zone to open, driving the membrane charge momentarily into a charge reversal (overshoot) of +35 mV, approaching the Nernst potential for sodium. At this point, the sodium channels commence a progressive inactivation, and the voltage-gated potassium channels are simultaneously triggered to open. Current flow switches from Na+ inflow to K+ outflow. The hyperpolarization phase is explained by the voltage-gated sodium channels being completely inactivated prior to closure of the potassium channels. Any remaining discrepancy is adjusted by activity of the Na+–K+ pump.
Close analyses of the sodium channels involved have revealed a dual mechanism of operation, as indicated in Figure 7.10. In the resting state, an activation gate in the midregion of both Na+ and K+ channel pores is closed. The sodium channel is the first to respond at threshold, by opening its activation gate and allowing a torrential inflow of Na+ ions down the concentration gradient. One millisecond later, a second, inactivation gate, in the form of a flap of globular protein, seals the exit into the cytosol while the K+ channel pore is opening. When the membrane potential approaches normality, the sodium gating reverts to its resting inactive state.
During the rising and early falling phases of the action potential, the neuron passes through an absolute refractory period where it is incapable of initiating a second impulse because too many voltage-gated channels are already open (Figure 7.11). This is followed by a relative refractory period, where stimuli in excess of the standard 15-mV requirement can elicit a response. It is quite common for the generator potential to reach up to 35 mV, triggering impulses at 50–100 impulses per second, expressed as 50–100 Hz (Hertz = times per second).
Propagation
Reversal of potential at the trigger zone is propagated (conducted) along the axon in accordance with the electrotonic circuit shown in Figure 7.12. The positive internal membrane charge passes in both directions within the axoplasm, while positive outside charge passes in both directions within the ECF to neutralize the negative external potential. The membrane immediately proximal is sufficiently refractory to resist depolarization, whereas that immediately distal undergoes a local response (depolarization) progressing to firing level. This process continues distally along the stem axon and its branches, thereby conducting the action potential all the way to the nerve terminals.
Conduction velocities
Myelinated nerve fibers range in external diameter (i.e. including the myelin sheath) from 2 to 25 µm. In addition to the two axonal size benefits mentioned, wider myelinated fibers possess longer internodal myelin segments. The spikes are accordingly further apart, with increased conduction velocity reminiscent of a runner with a longer stride. Conduction velocities for various kinds of peripheral nerve fibers are given in Chapter 9.