Chapter 9 Digital/Fast SPECT
Systems and Software
INTRODUCTION
Single-photon emission computed tomography (SPECT) myocardial perfusion imaging (MPI) has become a widely used and well-established medical imaging test. However, it suffers from some fundamental limitations that include long image acquisition, low image resolution, and patient radiation dose. Scan time is generally on the order of 15 to 20 minutes for each stress and rest acquisition, resulting in long overall test times and frequent artifacts from patient motion during the scan. Reduction of imaging times has been long recognized as an important factor in reducing patient motion artifacts and increasing throughput. Various efforts have been taken to reduce this time with standard equipment and cameras.1–3 Furthermore, the recent increase in the use of coronary computed tomography angiography (CCTA) in patients who also have SPECT MPI studies has intensified the concern about the levels of radiation associated with SPECT.4 Some of these limitations are intrinsically linked to each other; longer acquisition times could be used with lower injected doses, and higher doses could be used to shorten acquisition times.
DEDICATED CARDIAC IMAGING SYSTEMS
Several new dedicated hardware camera systems that have been introduced by various vendors for cardiac imaging attempt to improve imaging parameters primarily by optimizing acquisition geometry, collimator design, and reconstruction software.5 Innovative designs of the gantry and detectors have been introduced that allow increased sampling of the myocardial region and thus allow better local sensitivity for MPI imaging. The primary goals for the development of these systems are twofold: (1) to achieve improved spatial resolution and sensitivity and therefore allow for faster imaging times and (2) to improve patient comfort by permitting imaging in an upright or reclining position, eliminating the need to position the patient’s arms above the head. Furthermore, claustrophobic effects have been reduced or eliminated by technologies that reduce the size of the detector geometries and the associated mechanical structures. Overall sizes of the systems have also been reduced so they can be placed in locations with minimal available floor space. As a consequence of faster imaging times and more comfortable patient positioning, these systems have the additional benefit of reducing patient motion during a scan. In this section, we describe various available hardware designs and related reconstruction techniques.
Digirad Cardius 3 XPO
The first of these systems to be introduced was the Cardius XPO line manufactured by Digirad Corporation (Poway, CA). Although the initial design of a similar Digirad system was described in 1998 with the use of cadmium zinc telluride (CdZnTe, or CZT),6 all manufactured systems use solid-state cesium iodide (CsI)-photodiode detectors. This system can be configured in 1-, 2-, or 3-detector configurations.7 The Cardius 3 XPO is shown in Figure 9-1. Current models make use of pixilated CsI(Tl) detectors and photodiodes to configure detector heads that are more compact than conventional cameras equipped with photomultipliers. Each detector head is 21.2 × 15.8 cm and contains an array of 768 6.1 × 6.1 × 5 mm-thick CsI(Tl) crystals coupled to individual silicon photodiodes that are used to convert the light output of the crystals to electrical pulses. Digital software logic is used to process the signals and create images instead of analog Anger positional circuits. In the three-detector system, the detector heads are positioned at 67.5 degrees between heads, as shown in Figure 9-2. Heads are allowed to be moved in and out (closer to or farther away from the patient). For imaging, the patient sits on a chair with his arms placed on an armrest above the detectors. Data acquisition is typically accomplished in 7.5 minutes by rotating the patient chair by 67.5 degrees, producing a total acquisition arc of 202.5 degrees. With this system, the manufacturer reports a reconstructed spatial resolution of 8.95 mm (at a 20-cm orbit radius) and a sensitivity of 234 cpm/μCi, using the system’s cardiac collimator and a three-dimensional (3D) version of the ordered subset expectation maximization (OSEM) approach for reconstruction. Images of a patient with an inferior-wall defect acquired with this system are shown in Figure 9-3. These systems are now used clinically in several sites, and preliminary reports have been published comparing their performance to that of a standard dual-headed camera when it was found that similar quality could be obtained with a 38% reduction in acquisition time.8
Preliminary data have recently shown that the acquisition time can be further reduced with this scanner by the application of optimized image reconstruction protocols developed by Digirad. The nSPEED reconstruction9 models the depth-dependent detector spatial response of the SPECT systems with a 3D version of the OSEM reconstruction method. In preliminary reports,10 the image quality improvement with nSPEED, compared to a conventional two-dimensional OSEM technique,11 enables the reduction of acquisition time by 50% while maintaining image quality and information.
CardiArc
CardiArc Limited (Canton, MI) has developed a dedicated nuclear cardiology SPECT camera in which the detector and collimator are redesigned and optimized specifically for cardiac imaging.12 This device has no visibly moving parts but has a single internally moving part, which is hidden from the patient.13 Therefore, from the outside, the detector appears motionless, and for comfort the patient can be positioned upright. Scan times reported by the company are as short as 2 minutes.12 The camera system and a typical patient position are shown in Figure 9-4. This system was originally designed to use arrays of CZT crystals as detectors. However, owing to the high cost of CZT material and potential long-term stability issues with CZT,14 the detector design was changed to enable commercial production. Figure 9-5 illustrates the design and the principle of operation of the current model. The system incorporates a high-resolution detector with three curved sodium iodide–thallium (NaI[Tl]) crystals with graduated grooving technology and an array of 60 photomultiplier tubes arranged in three rows (see Fig. 9-5A). The gantry uses a proprietary digital process developed by CardiArc that replaces the conventional Anger logic. Horizontal photon collimation in each slice is accomplished using a thin, curved lead sheet with six narrow vertical slots (aperture arc; see Fig. 9-5A). Vertical slice collimation is accomplished using a series of stationary lead vanes that are stacked vertically between the aperture arc and the NaI(Tl) crystals (see Fig. 9-5B). In this way, data are collected as multiple 1 mm–thick slices using the six vertical apertures to collimate photons so they are detected continuously across the detector surface, with no overlap of data from different apertures. During acquisition, the aperture arc rotates to acquire data from multiple projections, providing 1280 angular samples in 0.14-degree increments over 180 degrees, which is an order of magnitude greater than the conventional camera angular sampling (typically 3 degrees). All detector pixels are active simultaneously, and photons can be detected from multiple angles (see Fig. 9-5C). The movement of the aperture arc is synchronized electronically with the areas of the NaI(Tl) crystals that are imaging the photons passing through the individual slots. The aperture arc’s weight of 35 pounds is lighter than traditional moving gantries, which facilities motion control. The aperture arc movement ranges 9 inches to cover the entire cardiac field of view, and each traverse of the arc takes 10 seconds.
SPECT reconstructed spatial resolution values (full-width half-maximum) quoted by CardiArc range from 3.6 mm (at 82 mm source-to-aperture arc distance) to 7.8 mm (at 337 mm source-to-aperture arc distance). An independent evaluation concluded that the CardiArc system appears to gain image quality by a factor of 5 to 10 when compared to the conventional dual-head camera.15 A comparison of patient imaging capabilities is shown in Figure 9-6.
Spectrum Dynamics
Spectrum Dynamics (Haifa, Israel) has manufactured a system called D-SPECT. The design and principle of its operation are shown in Figure 9-7. The patient is imaged in either a semireclining position with the left arm placed on top of the camera (see Fig. 9-7A) or in the supine position. Acquisition time as short as 2 minutes has been reported.16 This system uses pixilated CZT detector arrays (see Fig. 9-7B) mounted in nine vertical columns and placed in a 90-degree gantry geometry (see Fig. 9-7C). While CZT detectors are higher in cost, they have advantages of superior energy resolution (by a factor of approximately 1.7 at 140 keV) and compact size compared to the combination of NaI(Tl) with photomultiplier tubes of the conventional Anger camera. With D-SPECT, each detector column is fixed in a mechanical mounting, and the data acquisition is performed by rotating these multiple columns in synchrony. The photons from a given location are detected at multiple angles by multiple columns as the fields of view of the detectors are swept through the region of interest. Each column (see Fig. 9-7B) consists of an array of 1024 CZT elements (2.46 × 2.46 × 5 mm thick) arranged in a 16 × 64 element array with an approximate size of 40 × 160 mm. Each column is fitted with square parallel-hole high-sensitivity collimators, such that the dimensions of each hole are matched to the size of a single detector element. The collimators are fabricated from tungsten to eliminate the production of lead x-rays that might interfere with technetium-201 (201Tl) imaging. The collimators have a larger effective diameter than conventional low-energy high-resolution collimators used with scintillation cameras, yielding a significant gain in their geometric efficiency. The collimator has a hole length of 24.5 mm, with a 2.46-mm pitch and 2.26-mm hole diameter. The compensation for the loss in geometric spatial resolution that results from this design is accomplished by the software compensation methods. All data are collected in list mode. A proprietary Broadview iterative reconstruction algorithm based on the maximum-likelihood expectation maximization (MLEM) approach, with resolution recovery and use of the cardiac shape priors, has been developed and patented by the manufacturer.17 It allows the recovery of image resolution to 5 mm in line source experiments.
Data acquisition is accomplished in a two-step process. First, a 1-minute pre-scan is performed to identify the location of the region of interest. Scan limits and timings are then set for each detector column, and the final scan is performed with each detector column rotating within the limits set from the pre-scan data. This process is shown diagrammatically in Figure 9-8. This process is termed region-of-interest-centric scanning by the manufacturer, because the scan field is limited to only the myocardial region. It has not been possible to measure an absolute value of sensitivity for this system as prescribed by the National Electrical Manufacturers Association quality-control standards,18 because the sensitivity is significantly dependent on the field of view, defined individually for each patient by the pre-scan process. However, the most centrally located point has been reported to demonstrate a sensitivity of 1407 counts/μCi/min compared to the 160 to 240 counts/μCi/min range generally observed with standard cameras.19 A case example showing image quality on both D-SPECT and A-SPECT, obtained with the same isotope injection, is shown in Figure 9-9. In a recently published study, when D-SPECT was compared to A-SPECT, the myocardial count rate (with the same injection of the isotope) was 7 to 8 times higher for D-SPECT (Fig. 9-10).16 Preliminary work has shown that simultaneous dual-isotope SPECT MPI with this camera is feasible using 201Tl and technetium-99m, taking advantage of the improved energy resolution of CZT.20
The higher sensitivity of this system has been exploited to develop new clinical protocols. Cedars-Sinai Medical Center has reported the routine clinical use of this scanner in more than 400 patients using a stress 201Tl (2 mCi)/rest tetrofosmin or sestamibi (8 to 10 mCi) protocol. Using half of the radioactivity associated with standard dual-isotope procedures, this protocol includes upright and supine immediate poststress images of 6 minutes each followed by rest injection and immediate 4-minute rest imaging. The total imaging time of this protocol is 19 minutes. Good to excellent image quality without significant extracardiac interference was observed in over 96% of the cases.21
Multipinhole Systems
An alternative approach to standard parallel-hole collimation is multipinhole tomography. Previously, promising results with the multipinhole SPECT systems have been presented by several groups in small-animal imaging, surpassing the spatial resolution and detection efficiency of parallel-hole collimation.22–24 Because all views are active throughout the entire image acquisition period, SPECT imaging in this technique is accomplished without the need for motion of the detector, collimator, or patient. Since image acquisition is accomplished without any electromechanical motion, the manufacturing and servicing costs could potentially be significantly reduced.
The design of the cardiac system for human imaging was reported by Funk et al., with two 9-pinhole collimators attached to the standard dual-headed gamma camera,25 forming, in effect, an 18-pinhole imaging system. This cardiac imaging technology utilizes pinhole collimation to project a simultaneous set of images onto one or more large-area detectors. The principle of operation and a photograph of the actual prototype systems are shown in Figure 9-11. In experiments with the first prototype system, the authors found that spatial resolution of the 9-pinhole collimator with 8-mm diameter pinholes was 30% poorer than that for the parallel-hole collimator. However, the detection efficiency was increased by more than ten-fold. These data allowed them to predict that in comparison to a standard gamma camera, a five-fold increase in sensitivity could be achieved with this technology without degradation of image resolution. Similar increase in sensitivity has been demonstrated by the same group in the small-animal full-ring multipinhole SPECT system.26
Multipinhole design potentially suffers from some limitations that will need to be addressed. The approach may be prone to greater formation of artifacts, because it inherently produces an incomplete tomographic data set, and it acquires images from only limited views.25 Background activity from other organs may not be seen in all of the views, which could lead to inconsistencies in the reconstructed data. It is also known that the resolution and sensitivity of pinhole collimators decrease with the distance from the collimator27; however, resolution recovery can be applied during the reconstruction to compensate for this spatial variation. Nuclear Cardiology Research (NCR), located in Denver, Colorado, is currently commercializing this design. Original acquisition images are shown in Figure 9-12, and the reconstructed views are shown in Figure 9-13. The result of breath-by-breath motion correction is shown in Figure 9-14.
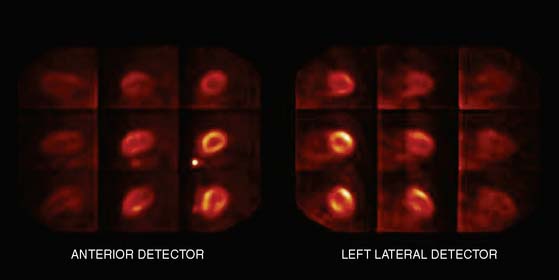
Figure 9-12 Original ungated raw views of myocardial perfusion obtained on a 9-pinhole system.
(Images courtesy of Dennis Kirch, Nuclear Cardiology Research, Denver, CO.)
FAST MYOCARDIAL PERFUSION IMAGING SOFTWARE WITH STANDARD SYSTEMS
Software improvements of image reconstruction have centered on the development of new proprietary algorithms that have evolved from the early work in iterative reconstruction techniques, MLEM,28,29 and later the accelerated method of OSEM.11 These techniques were developed to improve image contrast and reduce noise levels inherent in images with low counts reconstructed with FBP.
For purposes of comparison to the iterative methods, standard FBP reconstruction assumes that the object is detected equally in all of the angular projections. This leads to various artifacts caused by variations in attenuation, scatter, resolution, and count density. Iterative reconstruction methods based on MLEM and OSEM allow the geometry of the acquisition to vary for each projection, greatly enhancing the flexibility in modeling the physical parameters. SPECT imaging is greatly affected by Poisson noise, scatter, attenuation correction, and variable image resolution.30 The iterative methods make it possible to incorporate projection-specific corrections for these image-degrading factors into the reconstruction process so that the reconstructed image is a better representation of the object being imaged.
Currently, the most widely used iterative technique is based on the OSEM approach, which is an accelerated version of the MLEM algorithm and allows efficient computer processing. This technique groups projection data into an ordered sequence of subsets. One iteration of the OSEM algorithm is defined as a single pass through all of the subsets.11 Typically, 2 to 4 projections per subset are used with 4 to 12 iterations, which is computationally less demanding than 1 iteration of the standard MLEM algorithm (assuming 64 projections). Even with 1 iteration of OSEM and 32 subsets, it is possible to obtain a reasonable initial reconstruction. Typically, OSEM results in a degree of magnitude decrease of computing time without measurable loss of image quality, as demonstrated by Hudson and Larkin.11
OSEM techniques incorporate both backprojection and forward projection. Backprojection is the process of filling in a matrix by projecting back the data contained in the projection images. In this process, individual pixels in the reconstruction matrix are filled in along a ray corresponding to the direction from the projection data (Fig. 9-15). Forward projection is the reverse process: The data from the image reconstruction matrix are projected (summed) data from the image display matrix with movement out of the image matrix to form estimated projection data. Note also that MPI spatial resolution is a function of distance from the collimator, and that this can be modeled in the iterative reconstruction process (see Fig. 9-15).
In OSEM, reconstruction image data are updated during each iteration and for each subset. Therefore the number of updates is the product of iterations and projections subsets. As the number of updates increases, the spatial resolution increases, but the noise increases at the same time. This is demonstrated in Figure 9-16. This increase necessitates an optimization process where the noise-smoothing filter, the number of iterations, and the number of subsets are properly balanced to obtain optimal image quality—that is, spatial resolution and uniformity.
These iterative algorithms were extended to include depth-dependent and resolution recovery techniques, developed to correct for losses in spatial resolution due to the line response function of the collimator.31 Other physical effects that can be corrected are scatter and attenuation compensation. The algorithms currently available simultaneously address these problems by modeling the instrumentation and imaging parameters used for a specific application to eliminate the degrading effects of the line spread function (LSF) and to suppress noise in the image reconstruction process. The resolution recovery aspects of these algorithms can be emphasized to provide significant improvements in spatial resolution and image quality of SPECT sets, and the noise suppression aspects can be emphasized to permit decreased imaging times for SPECT acquisitions.
Astonish
The corrections for variations in spatial resolution use measurements of the changes in spatial resolution with distance from the collimator, as is shown in a general case in Figure 9-15. Calibrations for each of the collimators are measured initially by the manufacturer. Astonish software incorporates this collimator information into both the backprojection and the forward projection parts of the reconstruction. The resolution recover correction in Astonish can be performed with or without attenuation correction.
In Astonish, the corrections for the photon scatter are performed by the ESSE method described by Kadrmas et al.32 The corrections for the photon scatter are performed prior to the attenuation correction in each iterative OSEM step. Corrections for attenuation are performed during the forward projection process. Attenuation correction requires knowledge of both the photon attenuation coefficient and the density of each pixel that the “counts” are forward projected through. The density information is accessed in an attenuation map, modified from a previously acquired density image, with either a scanning line source or from a CT scanner.
One problem that all iterative methods must address is amplification of statistical noise during the reconstruction process. Astonish uses a proprietary (patent pending) noise-reduction method of smoothing both the estimated projection data and the measured projection data internally during the reconstruction process.33,34 This modification of OSEM allows for optimized control of Poisson image noise while maintaining higher image resolution. In addition, a Hanning prefilter (or optionally no filter) is used to smooth the projection images prior to starting reconstruction. The estimated projections are also smoothed with the same filter prior to the measured/estimated comparison being taken during each subset. This approach can be compared with other methods that smooth the image data after the reconstruction process.35
The clinical performance of Astonish has been tested in a multicenter trial consisting of 221 patients, and preliminary results have been reported.36 Image quality, interpretative certainty, and diagnostic accuracy were evaluated by three “blinded” readers for standard reconstruction for full-time FBP, full-time Astonish (FTA) (64 projections, 20 to 25 sec/projection), and half-time Astonish (HTA) (32 projections, 20 to 25 sec/projection). The HTA data were obtained from the full-time data by using half of the original projections. Stress and rest perfusion image qualities (excellent/good) were 87.8%/83.3% (FBP), 97.7%/95.9% (FTA), and 96.8%/95.5% (HTA), respectively (P < 0.001). Interpretive certainty and diagnostic accuracy (Fig. 9-17) were the same for all these techniques. An example of MPI image quality achievable with Astonish is shown in Figure 9-18.
GE Healthcare’s Evolution Software
GE Healthcare (Waukesha, WI) has also developed a modification of the OSEM algorithm that incorporates resolution recovery named “Evolution for Cardiac” or OSEM-RR. Their approach includes modeling of the integrated collimator and detector response function (CDR) in an iterative reconstruction algorithm, and performs image resolution recovery37 based on these parameters. This technique has been described in detail in a recent publication by DePuey et al.38 The OSEM-RR modeling includes basic collimator geometric response function for round-hole-shaped collimators31,39 that can be applied with good approximation to hexagonal holes.
The CDR compensation technique utilized in OSEM-RR was developed at the University of North Carolina, Chapel Hill, and Johns Hopkins University by Tsui et al.37,39,40 It is accomplished by convolving the projected photon ray with the corresponding LSF during iterative projection and backprojection. The following parameters are accounted for and compensated: collimator hole and septa dimensions, intrinsic detector resolution, crystal thickness, collimator-to-detector gap, and projection-angle-specific center of rotation–to-collimator face distances. These collimator-specific data are embedded in the software in the form of lookup tables. Some of the relevant acquisition parameters (such as object-to-collimator distance) are obtained directly from the raw projection data.
Additionally, similar to other optimized reconstruction methods, OSEM-RR incorporates noise suppression, which is required since the resolution recovery during the iterative reconstruction process amplifies noise, which can lead to the formation of hot spots in the final image. A maximum a posteriori technique41 is incorporated to control image noise in the OSEM-RR design. A modified one-step-late algorithm with a Green prior42 is utilized. The specific parameters in these reconstructions are optimized separately for each clinical protocol and separately for gated and attenuation-corrected images. The last iteration is performed using a median root prior.43
Siemens Medical Solutions
Siemens has recently released its software (Flash3D) incorporating iterative fast OSEM reconstruction with 3D resolution recovery, 3D collimator and detector response correction, and attenuation and scatter compensation.44 SPECT cardiac acquisition protocols (CardioFlash) have been developed utilizing Flash3D, where the acquisition time can be reduced to between 33% and 50%, compared to the standard acquisition protocols with FBP reconstruction. An example of the image quality obtained with CardioFlash is shown in phantoms (Fig. 9-19) and in clinical images (Fig. 9-20). To date, it has been shown in phantom data in combination with a few clinical scans that Flash3D allows faster acquisition protocols but still provides sufficient myocardial uniformity and lesion detectability.44 Prospective clinical studies are currently being conducted to assess the performance of this technique with a protocol using 6-degree angular sampling, thus reducing acquisition time by 50%.
The computer reconstruction times of the 2007 release of Flash3D (Siemens OSEM reconstruction with 3D distance-dependent resolution recovery and optional scatter and attenuation corrections) were improved, and it is now possible to process an entire clinical gated cardiac data set in less then 1 minute on a standard workstation. High correlation (r2 > 0.97) has been shown between the ejection fractions obtained from conventional FBP-based protocol and the CardioFlash reconstructions in a preliminary study.45
UltraSPECT Wide Beam Reconstruction
UltraSPECT Limited (Haifa, Israel) has developed a standalone workstation (Xpress.cardiac) that utilizes the patented wide beam reconstruction (WBR) algorithm.46 The WBR reconstruction technique, phantom validation, and its clinical application have been recently described by Borges-Neto et al.47 This solution is available as an additional workstation and can reconstruct data from most existing gamma cameras with standard collimator design. Similar to other methods, WBR models the physics and geometry of the emission and detection processes and attempts resolution recovery. During the iterative reconstruction, it corrects reconstructed voxels, given the information regarding the collimator’s geometry (such as the dimensions and shape of holes or the septa thickness). Furthermore, the detector’s distance from the patient is considered. New gamma cameras provide this information automatically for each angular position from their body contour tracking systems used for orbit prescription. However, this distance can also be obtained by image-processing techniques and definition of the 3D patient body contour, from which the distance of the detector to the body at a given location can be calculated.47 In addition, this technique applies statistical modeling of the expected photon emission in order to suppress Poisson noise. The standard approach to overcome noise is the application of Fourier domain post filtering; however, Fourier filtering does not distinguish between the actual signal and noise at a given frequency. WBR instead regularizes the likelihood objective function with a combination of the Poisson and Gaussian distributions. This is accomplished by Fourier analysis of a projection to determine the approximate signal-to-noise ratio that is present in the acquired data and the selection of an optimal noise model to yield the appropriate balance between resolution and noise. An example of WBR image quality is shown in Figure 9-21.
Motion-Frozen Reconstruction
Another development related to the quality and therefore potential time reduction in image reconstruction is the “motion-frozen” processing of gated cardiac images, which eliminates blurring of perfusion images due to cardiac motion.48 This technique applies a nonlinear, thin-plate-spline warping algorithm and shifts counts from the whole cardiac cycle into the end-diastolic (ED) position. The “motion-frozen” images have the appearance of ED frames but are significantly less noisy, since the counts from the entire cardiac cycle are used. The spatial resolution of such images is higher than that of summed gated images. This technique has been successfully applied to SPECT and PET images. Figure 9-22 shows an example of a SPECT image reconstructed with motion-frozen technique. A significant improvement in image resolution can be observed when compared to standard summed images. The combination of such advanced approaches dedicated to cardiac imaging and the general advances in image reconstruction described could result in further gains in image quality.
1. DePuey E.G., Nichols K.J., Slowikowski J.S., Scarpa W.J., Smith C., Melancon S. Fast stress (8 minute) and rest (10 minute) acquisitions for Tc-99m sestamibi separate day SPECT. J Nucl Med. 1995;36(1):569-574.
2. Mazzanti M., Germano G., Kiat H., Friedman J., Berman D.S. Fast technetium 99m-labeled sestamibi gated single-photon emission computed tomography for evaluation of myocardial function. J Nucl Cardiol. 1996;3(2):143-149.
3. Germano G., Kavanagh P.B., Berman D.S. Effect of the number of projections collected on quantitative perfusion and left ventricular ejection fraction measurements from gated myocardial perfusion single-photon emission computed tomographic images. J Nucl Cardiol. 1996;3(5):395-402.
4. Einstein A.J., Henzlova M.J., Rajagopalan S. Estimating risk of cancer associated with radiation exposure from 64-slice computed tomography coronary angiography. JAMA. 2007;298(3):317-323.
5. Patton J.A., Slomka P.J., Germano G., Berman D.S. Recent technologic advances in nuclear cardiology. J Nucl Cardiol. 2007;14(4):501-513.
6. Butler J.F., Lingren C.L., Friesenhahn S.J., et al. CdZnTe solid-state gamma camera. IEEE Trans Nucl Sci. 1998;45(3 Part 1):359-363.
7. Babla H., Bai C., Conwell R. A triple-head solid state camera for cardiac single photon emission tomography (SPECT). Proc Soc Phot Opt Instrum Eng. 2006;6319:63190M.
8. Lewin H.C., Hyun M.C. [Abstract] A clinical comparison of an upright triple-head digital detector system to a standard supine dual-head gamma camera. J Nucl Cardiol. 2005;12(4):113.
9. Bai C., Conwell R., Babla H., et al: Improving Image Quality and Imaging Efficiency Using nSPEED, 2008. http://www.digirad.com/downloads_2007/nSPEED_white_paper.pdf Accessed 05/30/2008
10. Maddahi J., Mendez R., Chuanyong B., et al. [Abstract] Validation of a method for fast myocardial perfusion gated SPECT imaging. J Nucl Cardiol. 2007;14:16.
11. Hudson H.M., Larkin R.S. Accelerated image reconstruction using ordered subsets of projection data. IEEE Trans Med Imaging. 1994;13(4):601-609.
12. www.CardiArc.com, 2008 Accessed 05/30/2008
13. Madsen M.T. Recent Advances in SPECT Imaging. J Nucl Med. 2007;48(4):661-673.
14. Arlt R., Rundquist D.E. Room temperature semiconductor detectors for safeguards measurements. Nucl Instrum Methods Phys Res A. 1996;380(1):455-461.
15. O’ Connor M. Evaluation of the CardiArc dedicated cardiac system [unpublished independent evaluation]. Rochester, MN: Mayo Clinic, 2005.
16. Sharir T., Ben-Haim S., Merzon K., et al. High-speed myocardial perfusion imaging: Initial clinical comparison with conventional dual detector anger camera imaging. JACC Cardiovasc Imaging. 2008;1:156-163.
17. Rousso B., Nagler M., Rousso B., Nagler MRousso B., Nagler Ms, Spectrum Dynamics LLC, assignee: Multi-dimensional image reconstruction, 2007. US patent 7176466. Feb 13, 2007
18. Hines H., Kayayan R., Colsher J., et al. Recommendations for implementing SPECT instrumentation quality control. Eur J Nucl Med Mol Imaging. 1999;26(5):527-532.
19. Patton J., Sandler M., Berman D., et al. D-SPECT: [Abstract] A new solid state camera for high speed molecular imaging abstract. J Nucl Med. 2006;47:189.
20. Ben-Haim S., Hutton B., Van Gramberg D., et al. Simultaneous dual isotope myocardial perfusion scintigraphy (DI MPS): Initial experience with fast D-SPECT [Abstract]. J Nucl Med. 2008;49(Suppl 1):72P.
21. Berman D.S., Kang X., Tamarappoo B., et al. Stress thallium-201/rest technetium-99m sequential dual isotope high-speed myocardial perfusion imaging. JACC Cardiovasc Imaging. 2009;2:273-282.
22. Jaszczak R.J., Li J., Wang H., Zalutsky M.R., Coleman R.E. Pinhole collimation for ultra-high-resolution, small-field-of-view SPECT. Phys Med Biol. 1994;39:425-437.
23. Schramm N.U., Ebel G., Engeland U., Schurrat T., Behe M., Behr T.M. High-resolution SPECT using multipinhole collimation. IEEE Trans Nucl Sci. 2003;50(3):315-320.
24. Beekman F.J., Vastenhouw B. Design and simulation of a high-resolution stationary SPECT system for small animals. Phys Med Biol. 2004;49(19):4579-4592.
25. Funk T., Kirch D.L., Koss J.E., Botvinick E., Hasegawa B.H. A Novel Approach to Multipinhole SPECT for Myocardial Perfusion Imaging. J Nucl Med. 2006;47(4):595-602.
26. Funk T., Després P., Barber W.C., Shah K.S., Hasegawa B.H. A multipinhole small animal SPECT system with submillimeter spatial resolution. Med Phys. 2006;33:1259-1268.
27. Metzler S.D., Bowsher J.E., Smith M.F., Jaszczak R.J. Analytic determination of pinhole collimator sensitivity with penetration. IEEE Trans Med Imaging. 2001;20(8):730-741.
28. Shepp L.A., Vardi Y. Maximum likelihood reconstruction for emission tomography. IEEE Trans Med Imaging. 1982;1:113-122.
29. Lange K., Carson R. EM reconstruction algorithms for emission and transmission tomography. J Comput Assist Tomogr. 1984;8(2):306-316.
30. El Fakhri G., Buvat I., Benali H., Todd-Pokropek A., Di Paola R. Relative Impact of Scatter, Collimator Response, Attenuation, and Finite Spatial Resolution Corrections in Cardiac SPECT. J Nucl Med. 2000;41(8):1400-1408.
31. Metz C.E. The geometric transfer function component for scintillation camera collimators with straight parallel holes. Phys Med Biol. 1980;25(6):1059-1070.
32. Kadrmas D.J., Frey E.C., Karimi S.S., Tsui B.M.W. Fast implementation of reconstruction-based scatter compensation in fully 3D SPECT image reconstruction. Phys Med Biol. 1998;43(4):857-873.
33. Ye J., Song X., Zhao Z., Da Silva A.J., Wiener J.S., Shao L. Iterative SPECT Reconstruction Using Matched Filtering for Improved Image Quality. IEEE Nucl Sci Symp Conf Rec (2006). 2006;4:2285-2287.
34. Ye J., Shao L., Zhao Z., Durbin M.: Iterative Reconstruction with Enhanced Noise Control Filtering, 2007. WO Patent WO/2007/034,342
35. Van Laere K., Koole M., Lemahieu I., Dierckx R. Image filtering in single-photon emission computed tomography: principles and applications. Comput Med Imaging Graph. 2001;25(2):127-133.
36. Venero C.V., Ahlberg A.W., Bateman T.M., et al. Enhancement of Nuclear Cardiac Laboratory Efficiency: Multicenter Evaluation of a New Post-Processing Method with Depth-Dependent Collimator Resolution Applied to Full and Half-Time Acquisitions. J Nucl Cardiol. 2008.
37. Tsui B.M.W., Hu H.B., Gilland D.R., Gullberg G.T. Implementation of simultaneous attenuation and detector response correction in SPECT. IEEE Trans Nucl Sci. 1988;35(1):778-783.
38. DePuey E., Gadiraju R., Clark J., Thompson L., Anstett F., Shwartz S. Ordered subset expectation maximization and wide beam reconstruction “half-time” gated myocardial perfusion SPECT functional imaging: A comparison to “full-time” filtered backprojection. J Nucl Cardiol. 2008;15:547-563.
39. Tsui B.M.W., Gullberg G.T. The Geometric Transfer-Function for Cone and Fan Beam Collimators. Phys Med Biol. 1990;35(1):81-93.
40. Tsui B.M.W., Frey E.C., Zhao X., Lalush D.S., Johnston R.E., McCartney W.H. The importance and implementation of accurate 3D compensation methods for quantitative SPECT. Phys Med Biol. 1994;39(3):509-530.
41. Bruyant P.P. Analytic and iterative reconstruction algorithms in SPECT. J Nucl Med. 2002;43(10):1343-1358.
42. Green P.J. Bayesian reconstructions from emission tomography data using a modified EM algorithm. IEEE Trans Med Imaging. 1990;9(1):84-93.
43. Alenius S., Ruotsalainen U. Bayesian image reconstruction for emission tomography based on median root prior. Eur J Nucl Med Mol Imaging. 1997;24(3):258-265.
44. Vija A.H., Zeintl J., Chapman J.T., Hawman E.G., Hornegger J. Development of Rapid SPECT Acquisition Protocol for Myocardial Perfusion Imaging. IEEE Nucl Sci Symp Conf Record. 2006;3:1811-1816.
45. Zeintl J., Ding X., Vija A.H., Hawman E.G., Hornegger J., Kuwert T. Estimation accuracy of ejection fraction in gated cardiac SPECT/CT imaging using iterative reconstruction with 3D resolution recovery in rapid acquisition Protocols. IEEE Nucl Sci Symp Conf Rec. 2007;6:4491-4496.
46. UltraSPECT. www.UltraSPECT.com, 2008 Accessed 09/06
47. Borges-Neto S.P.R., Shaw L.K., et al. Clinical results of a novel wide beam reconstruction method for shortening scan time of Tc-99m cardiac SPECT perfusion studies. J Nucl Cardiol. 2007;14:555-565.
48. Slomka P.J., Nishina H., Berman D.S., et al. Motion-frozen” display and quantification of myocardial perfusion. J Nucl Med. 2004;45(7):1128-1134.