CHAPTER 1 Development of the Spine
The embryologic development of the human spine is an enormously complex process that is only partially understood. Differentiation of the pluripotent tissues of the embryo leads to early formation of a repetitive segmented vertebral structure. Because the embryo is exquisitely susceptible to malformation and developmental error, each step of formation is critical.1–4 Familiarity with these various steps can be helpful in understanding not only congenital syndromes, but also the possible developmental role concerning predisposition to some degenerative spinal processes, typically considered “wear and tear” conditions.5,6 The continuously expanding understanding of the genetic basis of life, with the genetics of spinal development no exception, has aided the understanding of these syndromes.7–13
Early Embryologic Spine Precursors: Day 17 to Week 4
The development of the human spine begins on the 17th day of gestation. This is within the triploblastic stage of the embryo, during which it is shaped as a disc (Figs. 1–1 and 1–2). On one side of the disc is the amnion cavity, and on the other is the yolk sac. On the dorsal layer (which is in contact with the amnion) of the disc, there are epiblastic cells that converge and invaginate into the disc to form the primitive pit or node. When embedded within the tissue, it forms a tubelike structure that extends craniad, “burrowing” deep to the embryonic disc along its ventral surface. The tube cavity is in continuity with the amniotic fluid. This extension is known as the notochordal tube.
The notochord lies ventral to the neural tube in the midline. Mesodermal tissues on either side of these structures condense to form longitudinal columns. By the 19th day, there are three distinct columns on either side of the midline: (1) medial paraxial columns, which give rise to the somites; (2) intermediate mesodermal columns, which form the urogenital organs; and (3) lateral mesodermal plates, which form the gut cavities. In considering the development of the spine, attention is focused on the medial paraxial columns. The juxtaposition to the intermediate columns may help explain, however, why abnormalities of the urogenital tract are frequently associated with vertebral anomalies.1
The somites are arranged in consecutive fashion along the dorsal aspect of the embryo. They are first formed in the rostral (or cranial) aspect of the embryo, continuing caudad to form 42 to 44 individual segments over a period of days where the medial paraxial columns previously existed. Because they are close to the dorsal surface, they are visibly apparent as a series of beaded elevations (Fig. 1–3).
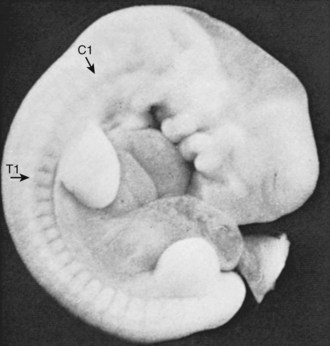
FIGURE 1–3 Somites of the human embryo are externally represented as a series of dorsolateral swellings.
Within the somite different regions have specialized fates (Figs. 1–4 and 1–5). The dorsolateral cells become the dermomyotomes. These eventually give rise to the skin (lateral) and muscle (medial) overlying the spine. The ventromedial cells within the somite become the sclerotomes. These are the precursors of the skeletal components (vertebrae) of the spine. The neural tube is fated to become the spinal cord.
From Somites to Spinal Column
Precartilaginous (Mesenchymal) Stage: Weeks 4 and 5
In metameric fashion, the sclerotomes are organized into a consecutively stacked arrangement. The next step in spinal development has been explained by the “resegmentation” theory.14–18 Resegmentation describes the division of each sclerotome into a cranial and caudal half. The cranial half is loosely arranged, whereas the caudal half is composed of densely packed cells. A small portion of the densely packed cells migrate superiorly to form the annular portion of the intervertebral disc, surrounding the notochord. Most of the densely packed cells fuse with the loosely packed cells of the adjacent caudal sclerotome. This fusion creates the centrum, the precursor of the vertebral body. The centrum develops from portions of two neighboring sclerotomes. This has significance on the anatomy of the fully developed spinal column. Initially, the segmental nerve precursors are located at the midportion of each sclerotome, whereas the segmental artery lies at the junction between two adjacent levels. After resegmentation, the nerve lies at the level of the disc and the artery lies at the mid-centrum, where one would expect to find them in the fully developed specimen.
Experimental data support the resegmentation theory.8,19–22 The crux of these experiments includes implanting a quail somite (from a quail embryo) within a chick embryo’s native somites. The quail somite is juxtaposed to a chick somite, and they develop together as the embryo grows. The quail tissue can be differentiated from the chick tissue using special staining techniques. Eventually, the somites give rise to sclerotomes that develop into centra. With the use of this model, it has been shown that the centrum does arise from the caudal and cranial halves of adjacent sclerotomes. The posterior arches (i.e., laminae) appear to follow this same pattern of growth.
This process seems to be highly influenced by the Pax1 and Pax9 genes.22 It is unclear whether the spinous process develops from one sclerotome or two adjacent levels.8,22 Other investigators have produced evidence of resegmentation using genetic labeling techniques.20 These studies involved injection of retroviral particles containing the lacZ transducing vector BAG into a single somite of a chick embryo. In other words, a single somite was genetically altered so that its cells would produce the lacZ gene product—the protein β-galactosidase. When the investigators evaluated the developed embryo, they detected β-galactosidase in the caudal and cranial halves of two adjacent vertebrae, suggesting that cells from the labeled somite were incorporated into two neighboring vertebrae.
Cartilaginous Stage: Weeks 6 and 7
Before the 6th week, the embryonic spinal precursor is composed of mesenchymal cells. Starting in the 6th week, cartilage-producing centers, or chondrification centers, form within each developing vertebra. Although type II collagen production within the extracellular matrix has been detected in the 5th week, it is most active during the cartilaginous stage; it tapers off during the ossification stage, but its production persists within the notochordal remnants of the nucleus pulposus.12 Two chondrification centers form in each half of the centrum, which eventually fuse into a solid block of cartilage. A hemivertebra is formed because of a failure of chondrification in one half of the vertebral body. The segmental arteries from either side of the centrum fuse at its middle aspect. Chondrification centers also form within each half of the vertebral arch and eventually fuse with each other in the midline and to the posterior aspect of the centrum.
Next, primitive cartilaginous transverse processes and spinous processes develop from the vertebral arch. More recent evidence has shown that the cartilaginous spinous process is formed from Msx1 and Msx2 (two embryologic proteins), producing mesenchymal cells, which require BMP4 to differentiate.23 These relationships highlight the important interactions of primordial proteins in governing further development of the spine.
Ossification Stage: Week 8 and Beyond
Primary ossification centers develop in utero. In the spine, ossification centers form within the cartilaginous template. There are three primary ossification centers in the typical embryonic vertebra: one in the center of the centrum and one in each of the vertebra arch halves. At about the 9th week, the preparation for ossification of the centrum is heralded by anterior and posterior excavations of the cartilaginous centrum produced by the invasion of pericostal vessels.24 These vessels produce ventral and dorsal vascular lacunae, which support the initial ossification (Fig. 1–6). Ossification of the centra starts first at the lower thoracic spine working craniad and caudad from that point.25
Secondary ossification centers develop after birth. In the spine, these appear after puberty. There are five centers: one in the tip of the spinous process, one in each transverse process tip, and one ring epiphysis in the superior and inferior endplates of the vertebral bodies. This development occurs at about 15 or 16 years of age, but eventually these ossification centers fuse in the middle of the 3rd decade (Fig. 1–7).26 The transverse processes of the lower cervical vertebrae, particularly C7, may show an additional costal center of ossification that produces the troublesome cervical rib; this reinforces the concept that all vertebrae primitively had the potential of forming ribs.
A pair of embryologic joints, known as neurocentral joints, is not present in the fully developed spine. These are located at the junction of the vertebral arches and the centrum but are anterior to the site of the future pedicle. Although not true “joints,” they allow expansion of the vertebral arch and spinal canal along with growth of the vertebral body. This expansion is most rapid between 18 and 36 weeks of gestation.2 At birth, the spinal canal diameter at L1 through L4 is approximately 70% of adult size, whereas at L5 it is only 50%.2 This indicates differential growth within regions of the vertebral column during fetal development. Full adult dimensions are reached by 1 year of life at L3 and L4 levels. The neurocentral joints persist until 3 to 6 years of age. The fusion of the fetal vertebral arches to the centra occurs well anterior to the pedicles, at the site of the neurocentral joints. The definitive vertebral body includes more than just the bone derived from the ossification center of the centrum, so the terms body and centrum are not accurately interchangeable (Figs. 1–8 and 1–9).
It is commonly thought that isthmic spondylolysis occurs because of a stress-type fracture within the pars interarticularis of the lower lumbar vertebrae, most commonly L5. Specific anatomic features of the adult lumbar spine, such as variation of the dimensions of the “lateral buttress” within the lumbar spine, have been described. Prenatal factors have been sought, but with limited success. Sagi and colleagues5 analyzed histomorphologically the lumbar spines of fetal spines aged 8 to 20 weeks to determine the sequence and location of ossification of the pars interarticularis of the various levels. They reported several findings: First, the pars begins to ossify in the 12th to 13th week of gestation. In the upper lumbar levels, ossification begins at the posterior portion of the pedicle and continues caudad, creating uniform ossification and trabeculation of the pars interarticularis. In contrast, the pars of the lower lumbar levels begins within the center of the pars itself, extending from this point to connect to the neighboring structures. Sagi and colleagues5 found that this resulted in uneven ossification. This finding may help explain areas of weakness within the pars interarticularis of the lower lumbar levels and may suggest that there is a prenatal predilection for a stress fracture in most individuals.
Fate of the Notochord
In the early embryo, the notochord serves as a rigid template around which the future vertebral column develops. It is a uniform structure that is present throughout the entire length of the primordial spinal column. A sheath exists around the notochord in its early stages. Immunohistochemical staining studies of 4- and 5-week embryos identified that a complex of extracellular matrix molecules is already present within this sheath, including sulfated glycosaminoglycans, hyaluronic acid, fibronectin, laminin, tenascin, and collagen II.27 Aggrecan, keratan sulfate, and other large aggregating proteoglycans (present in the mature spine) were not detected at this stage, suggesting that these appear later in development. The notochordal cells themselves showed reactivity to transforming growth factor-β, suggesting an early influence of this growth factor on the developing extracellular matrix milieu.27
In the 20-mm embryo, the notochord becomes an intrinsically segmented structure in the thoracic and lumbar region; in the 30-mm embryo, this structure is evident in the cervical region as well. Segmentation leads to areas of fusiform enlargements in the region of the intervertebral disc, while the notochord is slowly obliterated in the region of the developing vertebral bodies. Within the developing vertebral body, the notochord is stretched into a “mucoid streak” (see Fig. 1–5B). With continued growth, the mucoid streak disappears, leaving behind only bone.
The notochord expands in the region of the intervertebral disc to form the nucleus pulposus. This was originally described in detail by Luschka.28 The notochord is a major source of the nucleus pulposus, and it has been shown histochemically and autoradiographically that notochordal cells proliferate and remain vital several years after birth.29 Although notochordal cells generally do not seem to be demonstrable in the human nucleus pulposus of individuals older than 5 years of age, Schwabe30 reported their survival in the incarcerated discs of the sacrum in a series of specimens ranging from 22 to 45 years in age. A chordoma is abnormal neoplastic growth of notochord cells that remain within the spine in adult life. This suggests that notochordal rest cells can persist well into middle age in some individuals. These neoplasms may develop at any point along the original notochordal track but are usually in the rostral (basisphenoid or basiocciput) and caudal (sacral) regions.
As a theme of development of the spine, the region of the previous notochord lies anterior to the center of the fully developed vertebral body. This has been verified by Nolting and colleagues,25 who detected remnants of notochordal tissue anterior to the cartilaginous body center in 13 fetal spines aged 10 to 24 weeks. This finding further reinforces that using the terms centrum and vertebral body interchangeably is inaccurate.
From Neural Tube to Spinal Cord
On the 20th day, ectodermal tissues on either side of the neural plate become thick and “pucker up.” This area is known as the neural crest, which contains cells that eventually compose the neural elements. The mesenchymal tissue beneath the neural crest is the neural fold. As the folds grow toward the midline, the two neural crests meet and fuse on day 22. The underlying neural plate forms a tube, known as the neural tube, whose walls are composed of the previous neural plate. The neural tube invaginates itself within the dorsum of the embryo. On the 26th day, the fused neural crest cells invaginate into the embryo and divide into right and left globules. They are termed the dorsal root ganglia. They are oval and appear before ossification of the spine.31
Development of the Intervertebral Disc
The intervertebral disc warrants special attention because it is the pathologic focus of many spinal conditions. In the early stages of embryonic development, there are an increasing number of cells in the peripheral portion of the disc and a decreasing number adjacent to the notochord. As the embryo grows beyond a crown-rump length of 10 mm, the cells in the peripheral zone become elongated and are arranged in a lamellar pattern. When it reaches a length of 20 to 40 mm, collagen fibers begin to be synthesized and are exported from the cells, forming a collagen-rich extracellular matrix. The collagen fibers follow the pattern of the cells and are arranged in a lamellar pattern as well, giving the peripheral disc (or anulus fibrosus) its characteristic composition of circumferential bands of tissue. No fully continuous fibers span the entire periphery of the disc, but rather multiple strands interdigitate to create a highly tensile structure. These lamellar bundles appear to be more densely arranged in the anterior anulus and less dense in the posterior anulus of the developing spine, which may explain the propensity for posterior disc rupture in young patients.6 As the embryo passes into the fetal stage at 2 months, the cells begin to decrease in number, and the production of extracellular matrix is increased.
By the start of the fetal period, the disc has three distinct regions: (1) an external fibrous zone, (2) an internal hyaline zone surrounding the notochord, and (3) a fibrocartilaginous zone. The disc grows by interstitial and appositional growth.32 Interstitial growth refers to growth that occurs at the outer attachment of the anulus to the cartilaginous endplates. Appositional growth refers to growth that occurs longitudinally between the vertebra and the disc. Lamellar fibers form attachments to the cartilaginous endplates in the region of the nucleus pulposus, which completely encases the gel-like structure. The outer layers of the anulus become deeply embedded into the peripheral portion of the endplate cartilage.
There is a lack of agreement regarding the extent of the vascularity of the fetal intervertebral disc. In the fetal disc, the anulus pulposus seems to be vascularized. Taylor and Twomney33 found that a plexus of vessels around the circumference of the disc sent branches deep within the anulus. In contrast, Whalen and colleagues34 reported that these vessels entered only the outermost lamellae of the anulus fibrosus. In addition to vessels within the anulus, regularly spaced vascular channels within the cartilage have been shown within the interface between the cartilaginous endplate and the disc (Fig. 1–10). These channels most likely do not act as blood vessels but rather as a sinusoidal “cul-de-sac” system that delivers nutritional factors by diffusion. The deep regions of the disc are probably not vascularized at any point in development. The adult intervertebral disc is avascular, receiving nutrition only through diffusion through the endplates aided by the flux of fluid to and from the nucleus pulposus. This avascularity may be present at 17 to 24 weeks.24
Spinal Ligament Development
There is a paucity of literature concerning the development of the spinal ligaments in the human fetus. Misawa and colleagues35 dissected 25 human fetuses 6 to 24 weeks of age. They found that, at 6 to 7 weeks, “light zones” represented areas of low cell density that correlated with vertebral bodies, whereas “dark zones” were areas of high cell density and corresponded to the intervertebral regions. The posterior longitudinal ligament was first recognized at 10 weeks, whereas the appearance of the ligamentum flavum was concomitant with that of the lamina at 12 weeks. The fibers of the ligamentum flavum became discernible only at 15 weeks.
Influence of Fetal Movement
The importance of fetal spinal movement has been recognized only more recently. Boszczyk and colleagues36 used ultrasonography to study the movements of 52 normal fetal spines in utero. They found that rotational movements of 4 to 10 degrees were measurable in fetuses at 9 to 36 weeks. These investigators concluded that this amount of rotation influenced the ultimate morphology of the joint and that torsional stresses are present prenatally and postnatally. Functional demands on the spine may begin even before birth.
Occipitocervical Complex
Four occipital myotomes can be readily identified in the human embryo of 4-mm crown-rump length.37 The first is small, the second is of intermediate size, and the third and fourth are equivalent to the succeeding cervical segments. The first cervical nerve and the hypoglossal artery clearly delimit the most caudal occipital segment. Eight rootlets of the hypoglossal nerve can be discerned rostral to the hypoglossal artery, and these usually unite into four, but no less than three, main roots. This confirms the involvement of at least three precervical segments in the formation of the occiput. DeBeer38 claimed that a total of nine segments might be involved in skull formation. The first four appear very primitive but contribute to the preotic cranium, whereas the fifth is rudimentary, without a myotome. The last four segments are definite precursors of the occipital complex.
Atlantoaxial Complex
The axis and atlas, although considered two vertebral levels in the fully developed spine, actually arise from three different centra. Sensenig39 first described this in detail in 1937, and later O’Rahilly and Meyer40 provided a description. These three centra have been named the X, Y, and Z components. The apical X component at first projects into the early foramen magnum and forms an occipitoaxial joint. It has come to be known as the proatlas and constitutes the main portion of the odontoid process. Although it is commonly written that the odontoid process develops from the centrum of C1, this is probably not entirely true.41 Remains of the occipitocervical syndesmosis are apparent by the formation of the alar ligaments. The Y component becomes the centrum of the atlas, and the Z component becomes the centrum of the axis (C2). The X, Y, and Z components are related to the first, second, and third cervical nerves, which explains the redundancy of the numbering of the upper cervical nerves. In a more recent study, Muller and O’Rahilly42 determined that these three components actually develop from only two and a half sclerotomes in the chick embryo.
The most frequent manifestation of variant segmentation is the appearance of a third (midline) occipital condyle, also known as a basilar tubercle. This structure occurs as a projection on the basion (anterior central point) of the foramen magnum. Sometimes it is expressed as a simple rounded tubercle, but in better developed cases there is actually an articular facet that receives the tip of the odontoid process forming a true diarthrosis (joint). Occasionally, accessory facets lateral to the central projection are present. In a series of 600 skulls, some suggestion of a third condyle was present in 14% of specimens.43
Toro and Szepe44 observed that the third condyle often occurs with occipitalization of the atlas. They also thought that it may be the expression of the hypochordal arch of the “ante-proatlas.” As they used this term, it seems to designate the most caudal occipital somite (Fig. 1–11). A more complete separation of this ante-proatlas may form a true occipital vertebra. First described by Meckel in 1815, this malformation forms a more or less complete ring inferior to the foramen magnum, and its anterior arch is often fused to the skull, bearing a third condyle. This condition is distinguished from occipitalization of the atlas by the radiologic identification of the true atlas beneath it. Transverse processes of variable relative size may be present in occipital vertebrae, but these do not show a transverse foramen.45 Because bony eminences on either side of the third condyle are common to these structures, they may encroach on the foramen magnum, causing neurologic sequelae.
Occipitalization of the atlas occurs in 0.1% to 0.8% of the population according to the series of skulls examined. If the occipitalization is complete, there is no movable atlanto-occipital articulation, and the atlas ring is more constricted. Also, the level of the odontoid tip shows a higher relative position, and the fusion is often asymmetrical. Inglemark’s46 series of skulls showed that in 78% of the true congenital cases the posterior arch was fused to the posterior rim of the foramen magnum; the anterior arch was fused in 54%, and lateral fusions occurred in 23%. Toro and Szepe44 suggested that the variable expressions of fragments of the proatlas arch, which normally form parts of the atlas, may enhance the predilection of this segment to fuse to the skull.
Nonfused “floating” ossicles may occur within the craniocervical syndesmoses. A variably shaped, usually pea-sized, ossification that occurs between the basion and the tip of the odontoid (in the presence of a complete odontoid process) has been labeled Bergmann ossicle (Fig. 1–12)47 and is most likely a variant derivative of the ante-proatlas mesenchyme. Putz48 also recorded the incidence of a small ossicle between the anterior lip of the foramen magnum and the anterior arch of the atlas and within the anterior atlanto-occipital membrane. He was convinced that this was a manifestation of the hypochordal potential of the last occipital (ante-proatlas) somite.
Sacrum
Ossification of the bodies of the sacral vertebrae is unique in that, in addition to the single central ossific zone, two true epiphyseal plates later provide accessory ossification to the superior and inferior surfaces of each segment. The central centers for the superior three sacral vertebrae are evident at week 9, whereas these centers for the fourth and fifth segments do not appear until after week 24. Each vertebral arch of the sacrum shows the conventional bilateral centers, but in addition six centers produce the sacral alae. Between weeks 24 and 32, these centers appear anterolateral to the anterior sacral foramina of the upper three sacral vertebrae. They are expressions of the ever-present potential of the vertebral anlagen to produce costal equivalents (Fig. 1–13).
Genetic Control of Spinal Segmentation
Only after the segmental boundaries have been established can the structures characteristic of each segment be determined. These designations are effected by the homeotic selector genes. The term homeotic (from the Latin homoeos, meaning “similar”) was originally used by Bateson49 to label the mutant substitution of segment appendages because he surmised that they indicated a similarity (genetic homology) in their underlying developmental mechanisms. In contrast to the segmentation genes whose mutations affect the whole segment, mutations of the homeotic genes are expressed as homologous structures (e.g., legs and wings) grotesquely appearing on inappropriate segments. It is now known that these homeotic genes are closely grouped in two locations on the third chromosome of Drosophila’s four chromosomes.
Another significant outcome of Drosophila genetic research has been the identification of a sequence of nucleotide base pairs that is common to the homeotic selector genes.10 Intergenetic cross homologies of certain gene regions are not unusual, but the relatively small sequence common to these homeotic genes contained only a 180 base-pair unit that could easily be used as a probe to identify the locations of its homologues. This compact genetic fragment was called the homeobox by McGinnis and associates,50 and the protein it encodes is known as the homeodomain.10
Links Between Fly and Human
As would be expected, the homeobox-containing genes discovered in humans9 do not act in exactly the same manner as they do in Drosophila because the types of segmental organization are quite different. Nevertheless, the nucleotide sequence cognates of the Drosophila homeobox genes found in mammals seem to have considerable influence in the early establishment of brainstem and spinal cord formation.13 As in the more primitive forms, malfunctions of the genes controlling the more fundamental aspects of segmentation most likely produce early lethal mutations. Because higher vertebrates do not have an autonomous larval stage, the occurrence of such mutations would be lost to general observation. Nevertheless, some gross errors of segmentation that may reach parturition do show genetic implication.
Congenital Syndromes: Genetic Evidence of Segmentation in Humans
Klippel-Feil Syndrome
In humans, congenital vertebral fusions, most commonly manifest in the various “types” of the Klippel-Feil syndrome, serve as a prime example of segmentation. Many instances of this syndrome seem to result from spontaneous mutations or individual teratogenic accidents in the early developmental sequences because most reports present single case histories without examination of the extended family and the family’s pedigree. Gunderson and colleagues11 provided substantial evidence, however, that many cases of Klippel-Feil syndrome are probands of a familial history of the condition. These authors provided the pedigrees of 11 probands. Of particular interest is their type II of the syndrome, which exhibits fusions limited to the cervical regions at C2-3 and C5-6. Gunderson and colleagues11 concluded that this disorder, which produced segmentation errors at consistent spine levels through several successive generations, strongly indicated a dominant mutant defect of a gene that controls these specific levels of segmentation.
Caudal Dysplasias
Another class of segmental spinal malformations that indicates genetic import is grouped under the generic term of caudal dysplasias.4 This malformation complex has proved to be heritable and has a marked association with maternal diabetes. From this complex, certain insights into genetic mechanisms of mammalian spinal development may be derived. That some degree of caudal segment regression is a natural phenomenon is shown by the reduction of the original postsacral somites from eight (±2) to four (±1) in normal human development. In more severe forms of lumbosacral agenesis, all vertebral elements as far cephalad as the upper lumbar region may fail to develop. The association with maternal diabetes has been attributed to a teratogenic effect of hyperglycemia because experimental elevations of blood glucose have produced varying degrees of caudal deficiencies in animals.48 Similar effects have been induced by various toxic insults during embryogenesis of the spine.
Because caudal agenesis is not a consistent occurrence in the offspring of diabetic mothers, a more complex genetic association has been suspected, particularly as diabetes mellitus and spine defects have been associated with human leukocyte antigen (HLA)–type histocompatibility genes.4 This inference has been supported by studies of the T-locus genes in the mouse. This locus apparently is a segment of the mouse chromosomes with a collection of genes that have a profound effect on spine development and other aspects of embryogenesis.51 There is evidence that a gene complex, functionally similar to the mouse T locus, may be operable in humans because an association between histocompatibility antigens of the HLA type and the inheritance of human spina bifida has been reported.52
The HLAs are controlled by a cluster of contiguous genes located on the human chromosome 6. As in the mouse T locus, each gene in this group has several alleles, and numerous serologically discrete forms of cell surface antigens may be coded by the gene complex. The total ensemble of the HLAs produced within an individual determines its HLA “personality.”52 The comparative evidence suggests that the HLA complex, because of its defined chromosomal localization, its coding for the antigen complex, and its effect on spine development, is a reasonable candidate for the human analogue of the mouse T locus.
In vertebrates, as in other forms of segmented animals, a definite sequence of genetically controlled events establishes the basic aspects of segment formation. When this has been accomplished, some analogue of the homeotic system of genes most likely determines the regional specializations of the individual segments. This system provides an early determination within the vertebrate sclerotome because these embryonic cell masses exhibit a marked “position effect” before any regional differentiation of the somite is visibly evident. This effect has been shown in the chick embryo, in which the transplantation of an early thoracic sclerotome into the cervical region results in a rib-bearing thoracic vertebra whose specific character development was not modified by its heterotopic location.53 This early position identity may be because vertebrate embryonic patterns are mostly established through early cell-to-cell interactions subsequent to cell cleavages, and these involve the antigen-mediated cell surface recognitions and adhesions as shown by the HLAs. Nevertheless, some analogues of the homeotic mechanisms in Drosophila, although differing in their modes of expression, must determine whether a given vertebra exhibits cervical, thoracic, or sacral characteristics.
The range of anomalies observed in the human spine well support the concept that regional vertebral specification may be the result of a homeotic type of selective repression. In addition to the obvious articulated ribs of the thoracic region, each human vertebral level shows some expression of the costal element potential, but it is usually incorporated as an immovable projection. Anomalous free or articulated rib components have been observed at virtually every vertebral level, including the sacrum and coccyx.54 The hypochordal potential may best indicate the existence of early segmental totipotency in the vertebrates, however. This component is normally expressed at only the C1 level in humans and in the caudal region in other mammals. If there is some interference in the normal control mechanisms, it may also arise at other levels because hypochordal elements have been observed to occur below the last normal vertebra in some cases of lumbosacral agenesis.
1 Aoyama H, Asamoto K. The development fate of the rostral/caudal half of a somite for vertebra and rib formation: Experimental confirmation of the resegmentation theory using chick-quail chimeras. Mech Dev. 2000;99:71-82.
2 David KM, McLachlan JC, Aiton JF, et al. Cartilaginous development of the human craniovertebral junction as visualized by a new three-dimensional computer reconstruction technique. J Anat. 1998;192:269-277.
3 Misawa H, Ohtsuka K, Nakata K, et al. Embryological study of the spinal ligaments in human fetuses. J Spinal Disord. 1994;7:495-498.
1 Rai AS, Taylor TK, Smith GH, et al. Congenital abnormalities of the urogenital tract in association with congenital vertebral malformations. J Bone Joint Surg Br. 2002;84:891-895.
2 Ursu TR, Porter RW, Navaratnam V. Development of the lumbar and sacral vertebral canal in utero. Spine. 1996;21:2705-2708.
3 Wakimoto BT, Turner FR, Kaufman TC. Defects in embryogenesis in mutants associated with the antennapedia gene complex of Drosophila melanogaster. Dev Biol. 1984;102:147-172.
4 Welch JP, Alterman K. The syndrome of caudal dysplasia. Pediatr Pathol. 1984;2:313-327.
5 Sagi HC, Jarvis JG, Uhtoff HK. Histomorphic analysis of the development of the pars interarticularis and its association with isthmic spondylosis. Spine. 1998;23:1635-1639.
6 Tsuji H, Hirano N, Ohshima H, et al. Structural variation of the anterior and posterior annulus fibrosus in the development of human lumbar intervertebral disc: A risk factor for intervertebral disc rupture. Spine. 1993;18:204-210.
7 Akam ME. The molecular basis for metameric pattern in the Drosophila embryo. Development. 1987;101:1-22.
8 Aoyama H, Asamoto K. The development fate of the rostral/caudal half of a somite for vertebra and rib formation: Experimental confirmation of the resegmentation theory using chick-quail chimeras. Mech Dev. 2000;99:71-82.
9 Cannizzaro LA, Croce CM, Griffin CA, et al. Human homeobox containing genes located at chromosome regions 2q31-2q37 and 12q12-12q13. Am J Hum Genet. 1987;41:1-15.
10 Genring WJ, Hiromi Y. Homeotic genes and the homeobox. Ann Rev Genet. 1986;20:147-173.
11 Gunderson CH, Greenspan RH, Glasner GH, et al. The Klippel-Feil syndrome: Genetic and clinical reevaluation of cervical fusion. Medicine. 1967;46:491-511.
12 Krengel S, Gotz W, Herken R. Expression pattern of type II collagen mRNA during early vertebral development in the human embryo. Anat Embryol (Berl). 1996;193:43-51.
13 Tribioli C, Lufkin T. The murine Bapx1 homeobox gene plays a critical role in embryonic development of the axial skeleton and spleen. Development. 1999;126:699-711.
14 Baur R. Zum Problem der neugliederung der Wirbelsaule. Acta Anat. 1969;72:321-356.
15 Dalgleish AE. A study of the development of the thoracic vertebrae in the mouse assisted by autoradiography. Acta Anat. 1985;122:91-98.
16 Remak R. Untersuchungen uber die entwicklung der Wirbeltiere. Berlin: Riemer; 1855.
17 Verbout AJ. A critical review of the “Neugliederung” concept in relation to the development of the vertebral columns. Acta Biotheoret. 1976;25:219-258.
18 VonEbner V. Urwirbel und Neugliederung der Wirbelsaule. Sitzungber Akad Wiss Wein. 1889;III/101:235-260.
19 Bagnall KM, Higgins SJ, Sanders EJ. The contribution made by a single somite to the vertebral column: Experimental evidence in support of resegmentation using the chick-quail chimera model. Development. 1988;103:69-85.
20 Ewan KB, Everett AW. Evidence for resegmentation in the formation of the vertebral column using the novel approach of retroviral-mediated gene transfer. Exp Cell Res. 1992;198:315-320.
21 Huang R, Zhi Q, Brand-Saberi B, et al. New experimental evidence for somite resegmentation. Anat Embryol. 2000;202:195-200.
22 Huang R, Zhi Q, Neubuser A, et al. Function of somite and somitocele cells in the formation of the vertebral motion segment in avian embryos. Acta Anat. 1996;155:231-241.
23 Monsoro-Burq AH, Duprez D, Watanabe Y, et al. The role of bone morphogenetic proteins in vertebral development. Development. 1996;122:3607-3616.
24 Skawina S, Litwin JA, Gorczyca J, et al. The architecture of internal blood vessels in human fetal vertebral bodies. J Anat. 1997;191:259-267.
25 Nolting D, Hansen BF, Keeling J, et al. Prenatal development of the normal human vertebral corpora in different segments of the spine. Spine. 1998;23:2268-2271.
26 Noback CR, Robertson CC. Sequence of appearance of ossification centers in the human skeleton during the first five prenatal months. Am J Anat. 1951;89:1-28.
27 Gotz W, Osmers R, Herken R. Localisation of extracellular matrix components in the embryonic human notochord and axial mesenchyme. J Anat. 1995;186:111-121.
28 Luschka H. Die Halbgelenke des Menshlichen Korpers. Berlin: Reimer; 1858.
29 Malinski J. Histochemical demonstration of carbohydrates in human intervertebral discs during postnatal development. Acta Histochem. 1958;5:120-126.
30 Schwabe R. Untersuchungen uber die Ruckbildung der Bandscheiben im Menschlichen Kreuzbein. Virchows Arch. 1933;287:651-665.
31 Khorooshi MH, Hansen BF, Keeling J, et al. Prenatal localization of the dorsal root ganglion in different segments of the normal human vertebral column. Spine. 2001;26:1-5.
32 Bohmig R. Die Blutgefassversorg ung der Wirbelbandscheiben das Verhalten des intervertebralen Chordasegments. Arch Klin Chir. 1930;158:374-382.
33 Taylor JR, Twomney LT. The development of the human intervertebral disc. In: Ghosh P, editor. The Biology of the Intervertebral Disc. Boca Raton, FL: CRC Press, 1988.
34 Whalen JL, Parke WW, Mazur JM, et al. The intrinsic vasculature of developing vertebral end plates and the nutritive significance to the intervertebral disc. J Pediatr Orthop. 1985;5:403-410.
35 Misawa H, Ohtsuka K, Nakata K, et al. Embryological study of the spinal ligaments in human fetuses. J Spinal Disord. 1994;7:495-498.
36 Boszczyk AA, Boszczyk BM, Putz RV. Prenatal rotation of the lumbar spine and its relevance for the development of the zygapophyseal joints. Spine. 2002;27:1094-1101.
37 Sensenig EC. The early development of the human vertebral column. Contr Embryol Carneg Inst. 1957;33:21-51.
38 DeBeer GR. The Development of the Vertebral Skull. Oxford: Oxford University Press; 1937.
39 Sensenig EC. The origin of the vertebral column in the deer-mouse, Peromyscus maniculatus rufinus. Anat Rec. 1943;86:123-141.
40 O’Rahilly R, Meyer DB. The timing and sequence of events in the development of the vertebral column during the embryonic period proper. Anat Embryol. 1979;157:167-176.
41 David KM, McLachlan JC, Aiton JF, et al. Cartilaginous development of the human craniovertebral junction as visualized by a new three-dimensional computer reconstruction technique. J Anat. 1998;192:269-277.
42 Muller F, O’Rahilly R. Occipitocervical segmentation in staged human embryos. J Anat. 1994;185:251-258.
43 Lang J. Clinical Anatomy of the Head Wilson R, Winstanley DP (trans). Berlin: Springer-Verlag. 1983.
44 Toro I, Szepe L. Untersuchungen uber die Frage der Assimilation und Manifestation des Atlas. Z Anat Entwickl. 1942;111:186-200.
45 Hadley LA. Atlanto-occipital fusion, ossiculum terminale and occipital vertebra as related to basilar impression with neurological symptoms. Am J Radiol. 1948;59:511-524.
46 Inglemark BE. Uber das Craniovertebrale Grenzgebiet beim Menschen. Acta Anat Suppl. 1947;VI:1-116.
47 Bergman E. Die Lehre von den Kopfverletzungen (cited by Lang J). Stuttgart: Enke; 1880.
48 Putz VR. Zur Manifestation der hypochordalen Spangen im cranio-vertebralen Grenzebiet beim Menschen. Anat Anz. 1975;137:65-74.
49 Bateson W. Materials for the Study of Variation Treated with the Especial Regards to Discontinuity in the Origin of Species. London: Macmillan; 1894.
50 McGinnis W, Garber RL, Wirz J, et al. A homologous protein-coding sequence in Drosophila homeotic genes and its conservation in other metazoans. Cell. 1984;37:403-408.
51 Bennett D. The T-locus of the mouse. Cell. 1975;6:441-454.
52 Check W. First data for human developmental genes. JAMA. 1977;238:2253-2254.
53 Keynes RJ, Stern CD. Mechanisms of vertebrate segmentation. Development. 1988;103:413-429.