Chapter 3 Development of the Nervous System
The entire nervous system and the special sense organs originate from three sources, each derived from specific cell populations of the early epiblast termed neural ectoderm. The first source to be clearly delineated is the neural plate, which gives rise to the central nervous system (CNS), the somatic motor nerves and the preganglionic autonomic nerves. The second source is from cells at the perimeter of the neural plate, which remove themselves by epithelial–mesenchymal transition from the plate just prior to its fusion as a neural tube. These are the neural crest cells, and they form nearly all the peripheral nervous system (PNS), including the somatic sensory nerves, somatic and autonomic ganglia, postganglionic autonomic nerves and adrenal and chromaffin cells. They also give rise to significant mesenchymal populations in the head. The third source is from ectodermal placodes, a group of cells that originate at the edge of the neural plate but remain in the surface ectoderm after neural tube formation, undergoing epithelial–mesenchymal transformation after the neural crest cells have started their migration. Ectodermal placodes contribute to the cranial sensory ganglia, the hypophysis, the inner ear and, by a non-neuronal contribution, the lens of the eye.
Neurulation
Primary neurulation begins at stage 9 (Fig. 3.1). Although the process is continuous spatially and temporally, it has been envisaged as four stages. It begins with local elongation of the ectoderm cells in a midline zone of the disc and their reorganization into a pseudostratified epithelium, the neural plate. This is followed by reshaping of the neural plate and bending of the plate into a neural groove. The latter is closed to form a neural tube bidirectionally from the midportion to its cranial and caudal ends. A continuous surface ectoderm forms dorsal to the tube.
Early Vesicles and Flexures of the Neural Tube
Prior to closure of the neural tube, the neural folds become considerably expanded in the head region—the first indication of a brain. After the rostral neuropore closes, these regional expansions form three primary cerebral vesicles (Fig. 3.2). The term ‘vesicle’ may be a misnomer, because it suggests an exaggerated view of these localized accelerations of growth in the wall of the brain. The bulging is not initially marked, and the vesicles are more like gently fusiform tubes. The three regions are the prosencephalon (forebrain), mesencephalon (midbrain) and rhombencephalon (hindbrain), the last being continuous caudally with the spinal cord. As a result of unequal growth of their different regions, the prosencephalon and rhombencephalon enlarge more than the mesencephalon and can be subdivided. The prosencephalon gives rise to a midline diencephalon and bilateral telencephalon; the rhombencephalon gives rise to the metencephalon and myelencephalon. A summary of the derivatives of the cerebral vesicles is given in Table 3.1.
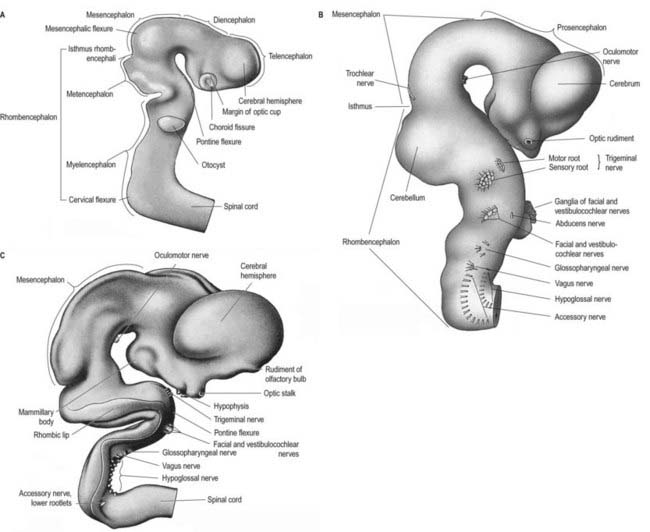
Fig. 3.2 A, Right side of the brain of a human embryo, 9 mm long. B, Right lateral surface of the brain of a human embryo, approximately 10.2 mm long. C, Right side of the brain of a human embryo, 13.6 mm long. The roof of the hindbrain has been removed.
Table 3.1 Derivatives of the cerebral vesicles from caudal to rostral
Rhombencephalon (or hindbrain) | |
1. Myelencephalon | Medulla oblongata |
Caudal part of the fourth ventricle | |
Inferior cerebellar peduncles | |
2. Metencephalon | Pons |
Cerebellum | |
Middle part of the fourth ventricle | |
Middle cerebellar peduncles | |
3. Isthmus rhombencephali | Superior medullary velum |
Superior cerebellar peduncles | |
Rostral part of the fourth ventricle | |
Mesencephalon (or midbrain) | Cerebral peduncles |
Tegmentum | |
Tectum | |
Aqueduct | |
Prosencephalon (or forebrain) | |
1. Diencephalon | Thalamus |
Metathalamus | |
Subthalamus | |
Epithalamus | |
Caudal part of the hypothalamus | |
Caudal part of the third ventricle | |
2. Telencephalon | Rostral part of the hypothalamus |
Rostral part of the third ventricle | |
Cerebral hemispheres | |
Lateral ventricles | |
Cortex (archaeocortex, palaeocortex, neocortex) | |
Corpus striatum |
Elongation of the brain occurs at the same time as the appearance of three flexures that are also developing prior to closure of the neural tube; two are concave ventrally, and one is concave dorsally. During stages 13 and 14 the brain bends at the mesencephalon (mesencephalic flexure), so the prosencephalon bends in a ventral direction around the cephalic end of the notochord and foregut until its floor lies almost parallel to that of the rhombencephalon (see Fig. 3.2). A bend also appears at the junction of the rhombencephalon and spinal cord (cervical flexure). This increases from the fifth to the end of the seventh week, by which time the rhombencephalon forms nearly a right angle to the spinal cord. However, after the seventh week, extension of the head takes place, and the cervical flexure diminishes and eventually disappears. The third bend, the pontine flexure, is directed ventrally between the metencephalon and myelencephalon. It does not substantially affect the outline of the head. In this region the roof plate thins until it is composed of only a single layer of cells and pia mater, the tela choroidea. The flexure of the neural tube at this point produces a rhombic shape in the roof that later forms the medullary velum.
In addition to these gross divisions, a number of ridges and depressions are present transiently on the inner surface of the brain. Prominent among these are the serial bulges that appear very early in the rhombencephalon, before the main flexures of the neural tube develop (Fig. 3.3). These bulges are termed rhombomeres.
Early Cellular Arrangement of the Neural Tube
Histologically, the early neural tube is composed of a pseudostratified neuroepithelium. It extends from the inner aspect of the tube to the outer limiting basal lamina and surrounding neural crest, which will form the pia mater. The epithelium contains stem cells that will give rise to populations of neuroblasts and glioblasts. A population of radial glia differentiates very early and provides a scaffold for later cells to follow. As development proceeds, three zones or layers develop (Figs. 3.4–3.6): an internal ventricular zone (variously termed the germinal, primitive ependymal or matrix layer), in which mitosis occurs and that contains the nucleated parts of the columnar cells and rounded cells undergoing mitosis; a middle mantle zone (also termed the intermediate zone), which contains the migrant cells from the divisions occurring in the ventricular zone; and an outer marginal zone, which initially consists of the external cytoplasmic processes of the radial glia and the neuroepithelial stem cells. The last is soon invaded by tracts of axonal processes that grow from neuroblasts developing in the mantle zone, together with varieties of non-neuronal cells (glial cells and later vascular endothelium and perivascular mesenchyme).
At first the neural tube caudal to the brain is oval in transverse section, and its lumen is narrow and slit-like (see Fig. 3.4). The original floor plate and the dorsal site of fusion of the tube initially contain non-neural cells. With cellular proliferation, the lateral walls thicken and the lumen, now the central canal, widens in its dorsal part and is somewhat diamond shaped on cross-section (see Fig. 3.6). Widening of the canal is associated with the development of a longitudinal sulcus limitans on each side. This divides the ventricular and mantle (intermediate) zones in each lateral wall into a ventrolateral lamina or basal plate and a dorsolateral lamina or alar plate. This separation indicates a fundamental functional difference.
Throughout the neural tube there is a generic pattern in the position of the neurones, specified by the juxtaposition of the notochord to the neural tube. Lateral or dorsal grafting of a notochord results in the induction of a floor plate overlying the grafted notochord and the induction of ectopic dorsal motor neurones. Similarly, lateral or dorsal grafts of a floor plate also result in the induction of a new floor plate overlying the graft and the induction of ectopic dorsal motor neurones. Removal of the notochord results in the elimination of the floor plate and motor neurones and the differentiation of dorsal cell types in the ventral region of the cord (Fig. 3.7).

Fig. 3.7 A–D, Successive stages in the development of the neural tube and spinal cord. A, The neural plate consists of epithelial cells. Cells in the midline of the neural plate are contacted directly by the notochord. More lateral regions of the neural plate overlie the paraxial mesenchyme (not shown). B, During neurulation, the neural plate bends at its midline, which elevates the lateral edges of the plate as the neural folds. Contact between the midline of the neural plate and the notochord is maintained at this stage. C, The neural tube is formed when the dorsal tips of the neural folds fuse. Cells in the region of fusion form the roof plate, which is a specialized group of dorsal midline cells. D, Cells at the ventral midline of the neural tube retain proximity to the notochord and differentiate into the floor plate. After neural tube closure, neuroepithelial cells continue to proliferate and eventually differentiate into defined classes of neurones at different dorsoventral positions within the spinal cord. For example, sensory relay, commissural and other classes of dorsal neurones (D) differentiate near the roof plate (R), and motor neurones (M) differentiate ventrally near the floor plate (F), which by this time is no longer in contact with the notochord (N). E–H, Summary of the results of experiments in chick embryos in which the notochord or floor plate is grafted to the dorsal midline of the neural tube or the notochord is removed before neural tube closure. E, The normal condition, showing the ventral location of motor neurones (M) and the dorsal location of sensory relay neurones (D). F, Dorsal grafts of a notochord result in induction of a floor plate in the dorsal midline and ectopic dorsal motor neurones (M). G, Dorsal grafts of a floor plate induce a new floor plate in the dorsal midline and ectopic dorsal motor neurones (M). H, Removal of the notochord results in the elimination of the floor plate and motor neurones and the expression of dorsal cells types (D) in the ventral region of the spinal cord.
(After Jessell, Dodd. 1992. WB Saunders.)
Failure of Neurulation
Failure of neurulation produces the conditions of craniorachischisis totalis (the entire neural tube is unfused in the dorsal midline), cranioschisis or anencephaly (the neural tube is fused dorsally to form the spinal cord but is not fused dorsally in the brain) and spina bifida (local regions of the spinal neural tube are unfused, or there is failure of formation of the vertebral neural arches) (Fig. 3.8). Anencephalic fetuses display severe disturbances in the shape, position and ossification of the basichondrocranium and in the course of the intracranial notochord (Fig. 3.9).
Neural Crest
The neuronal populations of the early epiblast are arranged in the medial region of the embryonic disc as the neural plate. Laterally, neural folds or crests indicate the transitional region between neural and surface ectoderm. Along most of the neuraxis the cells at the tips of the neural folds undergo an epithelial–mesenchymal transformation. They acquire migratory properties and leave the epithelium just prior to its fusion with the contralateral fold in the dorsal midline. The migratory cells so formed are collectively termed the neural crest (Brown, Keynes and Lumsden 2001). Cells within the rostral prosencephalic neural fold and smaller populations of cells in bilateral sites along the early brain do not form migratory neural crest cells but remain within the surface epithelium as ectodermal placodes.
Neural crest populations arise from the neural folds as primary neurulation proceeds and simultaneously progress rostrally and caudally. Crest cells migrate from the neural folds of the brain prior to tube closure. Caudally, from somite 27, secondary neurulation processes produce the most caudal neural crest. Two distinct populations of neural crest cells are formed: a neuronal population produced throughout the brain and spinal cord that gives rise to sensory and autonomic neurones and glia, and a non-neuronal mesenchymal population that arises only from the brain (Figs. 3.10, 3.11). Melanocytes develop from a subpopulation of neural crest cells derived from both the head and the trunk. They form one of the three pigment cell types (the others being retinal pigment epithelium and pigment cells of the pineal organ, both of which originate from the diencephalon).
In the trunk the migration patterns of neural crest cells are channelled by the somites. As the crest cells move laterally and ventrally they can pass between the somites and within the rostral sclerotomal half of each somite, but they cannot penetrate the caudal moiety of the sclerotomal mesenchyme. Thus the segmental distribution of the spinal and sympathetic ganglia is imposed on the neural crest cells by a prepattern that exists within the somitic paraxial mesenchyme (Fig. 3.12).
Rostral to the otic vesicle, neural crest cells arise from specific regions of the brain. Within the rhombencephalon a number of transverse subdivisions perpendicular to the long axis of the brain can be seen early in development. These are termed rhombomeres (neuromeres) to note their segmental arrangement (Muller and O’Rahilly 1997). At stage 9, six primary rhombomeres can be seen. Up to 16 secondary segments can be identified at stage 14. Eight main rhombomeres are recognized extending from the midbrain–hindbrain boundary rostrally to the spinal cord caudally (see Fig. 3.3). Rhombomeres 8 and 7 give rise to crest cells that migrate into the fourth and sixth pharyngeal arches; rhombomere 6 crest invades the third pharyngeal arch. Rhombomere 4 crest migrates into the second arch, whereas rhombomeres 5 and 3 give rise to a very small number of neural crest cells that migrate rostrally and caudally to enter the adjacent even-numbered neighbours. Rhombomeres 1 and 2 produce crest that invades the first pharyngeal arch. In each case mesenchymal populations and the sensory and autonomic ganglia are formed from the crest cells.
Ectodermal Placodes
Prior to neural tube closure, the elevating neural folds contain two distinctive neuronal populations. The larger population of neural crest cells migrates from the neural epithelium prior to neural tube fusion. A smaller population of neuroepithelial cells becomes incorporated into the surface ectoderm after neural tube closure. These areas of neuroepithelium within the surface ectoderm are termed ectodermal placodes. Although the majority of the ectodermal placodes form nervous tissue, non-neurogenic placodes also occur (Begbie and Graham 2001). After an appropriate inductive stimulus, local clusters of placodal cells remove themselves from the surrounding surface ectoderm either by epithelial–mesenchymal transition or by invagination of the whole placodal region to form a vesicle beneath the remaining surface ectoderm. Neurogenic placodes undergo both processes. Paired non-neurogenic placodes invaginate to form the lens vesicles under the inductive influence of the optic vesicles.
The neural folds meet in the rostral midline adjacent to the buccopharyngeal membrane. This rostral neural fold does not generate neural crest but gives rise to the hypophysial placode (i.e. the future Rathke’s pouch), which remains within the surface ectoderm directly rostral to the buccopharyngeal membrane. The rostral neural fold also gives rise to the olfactory placodes (which remain as paired, laterally placed placodes) and to the epithelium of the nasal cavity (see Fig. 3.11).
Further caudally, similar neurogenic placodes can be identified and divided into three categories: ventrolateral or epibranchial, dorsolateral and intermediate (Fig. 3.13). The epibranchial placodes appear in the surface ectoderm immediately dorsal to the area of pharyngeal (branchial) cleft formation. The first epibranchial placode is located at the level of the first pharyngeal groove and contributes cells to the distal (geniculate) ganglion of the facial nerve; the second and third epibranchial placodes contribute cells to the distal ganglia of the glossopharyngeal (petrosal) and vagus (nodose) nerves, respectively. Generally these placodes thicken, and cells begin to detach from the epithelium soon after the pharyngeal pouches have contacted the overlying ectoderm. Concurrently the neural crest cells reach and move beyond these lateral extensions of the pharynx. Cells budding off placodes show signs of early differentiation into neurones, including the formation of neurites. Epibranchial placodes may have their origins in the neurones that innervate the taste buds in fishes.
Intermediate between the epibranchial and dorsolateral placodes are the profundal and trigeminal placodes, which fuse in humans to form a single entity. Prospective neuroblasts migrate from foci dispersed throughout the surface ectoderm lateral and ventrolateral to the caudal mesencephalon and metencephalon to contribute to the distal portions of the trigeminal ganglia.
Pituitary Gland (Hypophysis Cerebri)
The most rostral portion of the neural plate, which will form the hypothalamus, is in contact rostrally with the future adenohypophysis, in the rostral neural ridge, and caudally with the neurohypophysis, in the floor of the neural plate (see Fig. 3.11). After neurulation the cells of the anterior neural ridge remain in the surface ectoderm and form the hypophysial placode, which is in close apposition and adherent to the overlying prosencephalon.
Neural crest mesenchyme later moves between the prosencephalon and surface ectoderm, except at the region of the placode. Before rupture of the buccopharyngeal membrane, proliferation of the periplacodal mesenchyme results in the placode forming the roof and walls of a saccular depression. This hypophysial recess (Rathke’s pouch; Figs. 3.14, 3.15) is the rudiment of the adenohypophysis. It lies immediately ventral to the dorsal border of the buccopharyngeal membrane, extending in front of the rostral tip of the notochord and retaining contact with the ventral surface of the prosencephalon. It is constricted by continued proliferation of the surrounding mesenchyme to form a closed vesicle, but it remains connected for a time to the ectoderm of the stomodeum by a solid cord of cells that can be traced down the posterior edge of the nasal septum. Masses of epithelial cells form mainly on each side and in the ventral wall of the vesicle, and development of the adenohypophysis progresses by the ingrowth of a mesenchymal stroma. Differentiation of epithelial cells into stem cells and three differentiating types is apparent during the early months of fetal development. It has been suggested that different types of cells arise in succession and that they may be derived in varying proportions from different parts of the hypophysial recess. A craniopharyngeal canal, which sometimes runs from the anterior part of the hypophysial fossa of the sphenoid to the exterior of the skull, often marks the original position of the hypophysial recess. Traces of the stomodeal end of the recess are usually present at the junction of the septum of the nose and the palate. Some claim that the craniopharyngeal canal itself is a secondary formation caused by the growth of blood vessels and that it is unconnected to the stalk of the anterior lobe.
Just caudal to, but in contact with, the adenohypophysial recess, a hollow diverticulum elongates toward the stomodeum from the floor of the neural plate just caudal to the hypothalamus (see Fig. 3.15B); this region of neural outgrowth is the neurohypophysis. It forms an infundibular sac, the walls of which increase in thickness until the contained cavity is obliterated except at its upper end, where it persists as the infundibular recess of the third ventricle. The neurohypophysis becomes invested by the adenohypophysis, which extends dorsally on each side of it. The adenohypophysis gives off two processes from its ventral wall that grow along the infundibulum and fuse to surround it, coming into contact with the tuber cinereum and forming the tuberal portion of the hypophysis. The original cavity of Rathke’s pouch remains first as a cleft and later as scattered vesicles; it can be identified readily in sagittal sections through the mature gland. The dorsal wall of Rathke’s pouch, which remains thin, fuses with the adjoining part of the neurohypophysis as the pars intermedia.
At birth the hypophysis is about one-sixth the weight of the adult gland; it increases to become about one-half the weight of the adult gland at 7 years and attains adult weight at puberty. Throughout postnatal life the gland is both larger and heavier in females.
Neuroglia
The ventricular zone lining the early central canal of the spinal cord and the cavities of the brain gives rise to neurones and glial cells (see Figs. 3.4, 3.5). One specialized form of glial cell is the radial glial cell, whose radial processes extend both outward, to form the outer limiting membrane deep to the pia mater, and inward, to form the inner limiting membrane around the central cavity. The geometry of these cells may provide contact guidance paths for cell migrations, both neuroblastic and glioblastic. A secondary radial glial scaffold is formed in the late-developing cerebellum and dentate gyrus and serves to translocate neuroblasts, formed in secondary germinal centres, to their definitive adult locations. Radial glia eventually lose their connections with both inner and outer limiting membranes, except for those that persist in the retina as Müller cells, in the cerebellum as Bergmann glia and in the hypothalamus as tanycytes. They can differentiate into neurones as well as astrocytes. They may partially clothe the somata of neighbouring developing neurones (between presumptive synaptic contacts) or similarly enwrap the intersynaptic surfaces of their neurites. Glial processes may expand around intraneural capillaries as perivascular end-feet. Other glioblasts retain an attachment (or form new expansions) to the pia mater, the innermost stratum of the meninges, as pial end-feet. Glioblasts also line the central canal and cavities of the brain as generalized or specialized ependymal cells, but they lose their peripheral attachments. In some situations, such as in the anterior median fissure of the spinal cord, ependymal cells retain their attachments to both the inner and outer limiting membranes. Thus, glia function as perineuronal satellites and provide cellular channels interconnecting extracerebral and intraventricular cerebrospinal fluid, the cerebral vascular bed, the intercellular crevices of the neuropil and the cytoplasm of all neural cell varieties.
Mechanisms of Neural Development
For more than a century the mechanisms that operate during development of the nervous system have been studied experimentally. Although much has been established, answers to many fundamental questions still remain obscure. In recent years, significant advances in our understanding of the development of vertebrates have come from work on amphibian, chicken, mouse and fish embryos and from the production of embryonic chimera (Le Douarin, Teillet and Catala, 1998). A combination of genetic, embryological, biochemical and molecular techniques has been used to elucidate the mechanisms operating in early neural populations.
Histogenesis of the Neural Tube
The wall of the early neural tube consists of an internal ventricular zone (sometimes termed the germinal matrix) abutting the central lumen. It contains the nucleated parts of the pseudostratified columnar neuroepithelial cells and rounded cells undergoing mitosis. The early ventricular zone also contains a population of radial glial cells whose processes pass from the ventricular surface to the pial surface, thus forming the internal and external glia limitans (glial limiting membrane). As development proceeds, the early pseudostratified epithelium proliferates, and an outer layer (the marginal zone), devoid of nuclei but containing the external cytoplasmic processes of cells, is delineated. Subsequently, a middle mantle layer (the intermediate zone) forms as the progeny from the ventricular zone migrate ventriculofugally (see Fig. 3.5).
Most CNS cells are produced in the proliferative zone adjacent to the future ventricular system, and in some regions this area is the only actively mitotic zone. According to the monophyletic theory of neurogenesis, it is assumed to produce all cell types. The early neural epithelium, including the deeply placed ventricular mitotic zone, consists of a homogeneous population of pluripotent cells whose varying appearances reflect different phases in a proliferative cycle. The ventricular zone is considered to be populated by a single basic type of progenitor cell and to exhibit three phases. The cells show an ‘elevator movement’ as they pass through a complete mitotic cycle, progressively approaching and then receding from the internal limiting membrane (Fig. 3.16). DNA replication occurs while the cells are extended and their nuclei approach the pial surface; they then enter a premitotic resting period as the cells shorten and their nuclei pass back toward the ventricular surface. The cells now become rounded close to the internal limiting membrane and undergo mitosis. They then elongate, and their nuclei move toward the outer edge during the postmitotic resting period, after which DNA synthesis commences once more, and the cycle is repeated. The cells so formed may either start another proliferative cycle or migrate outward (i.e. radially) and differentiate into neurones as they approach and enter the adjacent stratum. This differentiation may be initiated as they pass outward during the postmitotic resting period. The proliferative cycle continues with the production of clones of neuroblasts and glioblasts. This sequence of events has been called interkinetic nuclear migration, and it eventually declines. At the last division, two postmitotic daughter cells are produced, and they differentiate at the ventricular surface into ependyma.
The progeny of some of these divisions move away from the ventricular zone to form an intermediate zone of neurones. The early spinal cord and much of the brain stem shows only these three main layers: ventricular, intermediate and marginal zones. However, in the telencephalon, the region of cellular proliferation extends deeper than the ventricular zone, where the escalator movement of interkinetic migration is seen, and a subventricular zone appears between the ventricular and intermediate layers (see Fig. 3.5). Here cells continue to multiply to provide further generations of neurones and glia, which subsequently migrate into the intermediate and marginal zones. In some regions of the nervous system (e.g. the cerebellar cortex) some mitotic subventricular stem cells migrate across the entire neural wall to form a subpial population and establish a new zone of cell division and differentiation. Many cells formed in this site remain subpial in position, but others migrate back toward the ventricle through the developing nervous tissue and finish their migration in various definitive sites where they differentiate into neurones or macroglial cells. In the cerebral hemispheres, a zone termed the cortical plate is formed outside the intermediate zone by radially migrating cells from the ventricular zone. The most recently formed cells migrate to the outermost layers of the cortical plate, so that earlier formed and migrating cells become subjacent to those migrating later. In the forebrain there is an additional transient stratum deep to the early cortical plate, the subplate zone.
Lineage and Growth in the Nervous System
Growth Cones
During development, the growing axons of neuroblasts navigate with precision over considerable distances, often pursuing complex courses to reach their targets. Eventually they make functional contact with their appropriate end-organs (neuromuscular endings, secretomotor terminals, sensory corpuscles or synapses with other neurones). During the outgrowth of axonal processes, the earliest nerve fibres are known to traverse appreciable distances over an apparently virgin landscape, often occupied by loose mesenchyme. A central problem for neurobiologists, therefore, has been understanding the mechanisms of axon guidance (Gordon-Weeks 2000). Axon guidance is thought to involve short-range local guidance cues and long-range diffusible cues, any of which can be either attractive and permissive for growth or repellent and inhibitory. Short-range cues require factors that are displayed on cell surfaces or in the extracellular matrix; for example, axon extension requires a permissive, physical substrate, the molecules of which are actively recognized by the growth cone. They also require negative cues that inhibit the progress of the growth cone. Long-range cues come from gradients of specific factors diffusing from distant targets, which cause neurones to turn their axons toward the source of the attractive signal. The evidence for this has come from in vitro co-culture studies. The floor plate of the developing spinal cord exerts a chemotropic effect on commissural axons that later cross it, whereas there is chemorepulsion of developing motor axons from the floor plate. These forces are thought to act in vivo in concert in a dynamic process to ensure the correct passage of axons to their final destinations and to mediate their correct bundling together en route.
Dendritic Tree
Once growth cones have arrived in their general target area, they have to form terminals and synapses. In recent years, much emphasis has been placed on the idea that patterns of connectivity depend on the death of inappropriate cells. Programmed cell death, or apoptosis, occurs during the period of synaptogenesis if neurones fail to acquire sufficient amounts of specific neurotrophic factors. Coincident firing of neighbouring neurones that have found the appropriate target region might be involved in eliciting the release of these factors, thus reinforcing correct connections. Such mechanisms may explain the numerical correspondence between neurones in a motor pool and the muscle fibres innervated. On a subtler level, pruning of collaterals may give rise to mature neuronal architecture. The projections of pyramidal neurones from the motor and visual cortices, for example, start out with a similar architecture; the mature repertoire of targets is produced by the pruning of collaterals, leading to loss of projections to some targets.
Induction and Patterning of the Brain and Spinal Cord
The regional pattern of the nervous system is induced before and during neural tube closure. Early concepts about regional patterning envisaged that regionalization within mesenchymal populations that transmit inductive signals to the ectoderm imposes a similar mosaic of positional values on the overlying neural plate. For example, transplantation of caudal mesenchyme beneath the neural plate in Amphibia induced spinal cord, whereas rostral mesenchyme induced brain, as assessed by the morphology of the neuroepithelial vesicles. However, later work indicated a more complex scenario in which organizer grafts from early embryos induced mainly head structures, whereas later grafts induced mainly trunk structures. Subsequent molecular data tend to support a model in which neural-inducing factors released by the organizer, such as noggin, chordin and follistatin, neuralize the ectoderm and promote a mainly rostral neural identity. Later secreted signals then act to caudalize this rostral neural tissue, setting up an entire array of axial values along the neural tube. Candidates for these later caudalizing signals include retinoic acid, FGFs, and the WNT secreted proteins, which are present in the paraxial mesenchyme and later in its derivatives, the somites. This combination of signals does not seem to be sufficient to produce the most rostral forebrain structures. Other secreted proteins resident in the rostralmost part of the earliest ingressing axial populations of endoderm and mesenchyme are also capable of inducing markers of forebrain identity from ectodermal cells (Withington, Beddington and Cooke 2001).
Segmentation in the Neural Tube
One mechanism involved in the process of regional differentiation of cell populations within the neural tube is segmentation, which is conspicuous in humans and other vertebrates in the serial arrangement of the vertebrae and axial muscles and in the periodicity of the spinal nerves. In the last century, the possibility that the neural tube might be divided into segments or neuromeres was entertained, but some contended that the bulges observed in the lateral walls of the neural tube were artifacts or were caused by mechanical deformation of the tube by adjacent structures. Recent years have seen a resurgence of interest in this subject and a detailed evaluation of the significance of neuromeres. A series of eight prominent bulges that appear bilaterally in the rhombencephalic wall early in development have been termed rhombomeres (see Fig. 3.3). (Whereas the term neuromere applies generally to putative ‘segments’ of the neural tube, the term rhombomere applies specifically to the rhombencephalon.) Many aspects of the patterning of neuronal populations and the elaboration of their axon tracts conform to a segmental plan, and rhombomeres have now been shown to constitute crucial units of pattern formation. Domains of expression of developmental control genes abut rhombomere boundaries, and perhaps most importantly, single-cell labelling experiments have revealed that cells within rhombomeres form segregated non-mixing populations (Fig. 3.17). The neural crest also shows intrinsic segmentation in the hindbrain and is segregated into streams at its point of origin in the dorsal neural tube. This may represent a mechanism whereby morphogenetic specification of the premigratory neural crest cells is conveyed to the pharyngeal arches. Although these segmental units lose their morphological prominence with subsequent development, they represent the fundamental ground plan of this part of the neuraxis, creating a series of semiautonomous units within which local variations in patterning can develop. The consequences of early segmentation for later developmental events, such as the formation of definitive neuronal nuclei within the brain stem, and of peripheral axonal projections remain to be explored.
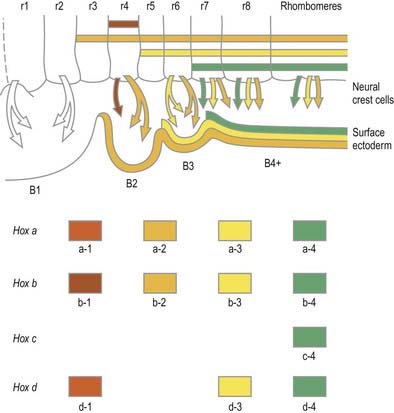
(Modified by permission from Annual Review of Cell and Developmental Biology, Vol. 8. 1992. www.annualreviews.org.)
Genes such as the Hox and Pax gene families, which encode transcription factor proteins, show intriguing expression patterns within the nervous system. Genes of the Hox-b cluster, for example, are expressed throughout the caudal neural tube and up to discrete limits in the hindbrain that coincide with rhombomere boundaries. The ordering of these genes within a cluster on the chromosome (5′–3′) is the same as the caudal-to-rostral limits of expression of consecutive genes. This characteristic pattern is surprisingly similar in fish, frogs, birds and mammals. Hox genes play a role in patterning of not only the neural tube but also much of the head region, consistent with their expression in neural crest cells and within the pharyngeal arches. Disruption of the Hox a-3 gene in mice mimics DiGeorge syndrome, a congenital human disorder characterized by the absence (or near absence) of the thymus, parathyroid and thyroid glands; hypotrophy of the walls of the arteries derived from the aortic arches and subsequent conotruncal cardiac malformations. Some Pax genes are expressed in different dorsoventral domains within the neural tube. Pax-3 is expressed in the alar lamina, including the neural crest, whereas Pax-6 is expressed in the intermediate plate. The Pax-3 gene has the same chromosomal localization as the mouse mutation Splotch and the affected locus in the human Waardenburg’s syndrome, both of which are characterized by neural crest disturbances with pigmentation disorders and occasional neural tube defects. Both Hox and Pax genes have restricted expression patterns with respect to the rostrocaudal and dorsoventral axes of the neural tube, consistent with roles in positional specification. (For reviews of the expression patterns of these genes, see Krumlauf et al 1993.)
Whereas craniocaudal positional values are probably conferred on the neuroepithelium at the neural plate or early neural tube stage, dorsoventral positional values may become fixed later. The development of the dorsoventral axis is heavily influenced by the presence of the underlying notochord. The notochord induces the ventral midline of the neural tube, the floor plate. This specialized region consists of a strip of non-neural cells with distinctive adhesive and functional properties. Notochord and floor plate together participate in inducing the differentiation of the motor columns. Motor neurone differentiation occurs early, giving some support to the idea of a ventral-to-dorsal wave of differentiation. The notochord–floor plate complex may also be responsible for allotting the values of more dorsal cell types within the tube (see Fig. 3.7). For example, the dorsal domain of Pax-3 expression extends more ventrally in embryos experimentally deprived of notochord and floor plate, whereas grafting an extra notochord adjacent to the dorsal neural tube leads to the repression of Pax-3 expression.
Peripheral Nervous System
Somatic Nerves
Spinal Nerves
Each spinal nerve is connected to the spinal cord by a ventral root and a dorsal root (Fig. 3.18). The fibres of the ventral roots grow out from cell bodies in the anterior and lateral parts of the intermediate zone. These pass through the overlying marginal zone and external limiting membrane. Some enter the myotomes of the somites, and some penetrate the somites, reaching the adjacent somatopleure; in both sites they ultimately form the α-, β- and γ-efferents. At appropriate levels these are accompanied by the outgrowing axons of preganglionic sympathetic neuroblasts (segments T1–L2) or preganglionic parasympathetic neuroblasts (S2–4).
The fibres of the dorsal roots extend from cell somata in dorsal root ganglia into the spinal cord and also into the periphery. Neural crest cells are produced continuously along the length of the spinal cord, but gangliogenic cells migrate only into the rostral part of each somitic sclerotome, where they condense and proliferate to form a bilateral series of oval-shaped primordial spinal ganglia (dorsal root ganglia; see Fig. 3.12). Negative factors in the caudal sclerotome deter neural crest from entering. The rostral sclerotome has a mitogenic effect on the crest cells that settle within it. From the ventral region of each ganglion, a small part separates to form sympathochromaffin cells, whereas the remainder becomes a definitive spinal ganglion (dorsal root ganglion). The spinal ganglia are arranged symmetrically at the sides of the neural tube and, except in the caudal region, are equal in number to the somites. The cells of the ganglia, like the cells of the intermediate zone of the early neural tube, are glial and neuronal precursors. The glial precursors develop into satellite cells (which become closely applied to the ganglionic nerve cell somata), Schwann cells and possibly other cells. The neuroblasts, which are initially round or oval, soon become fusiform, and their extremities gradually elongate into central and peripheral processes. The central processes grow into the neural tube as the fibres of dorsal nerve roots, and the peripheral processes grow ventrolaterally to mingle with the fibres of the ventral root, thus forming a mixed spinal nerve. As development proceeds, the original bipolar form of the cells in the spinal ganglia changes, and the two processes become approximated until they ultimately arise from a single stem to form a unipolar cell. The bipolar form is retained in the ganglion of the vestibulocochlear nerve.
Cranial Nerves
Cranial nerves may contain motor, sensory or both types of fibres. With the exception of the olfactory and optic nerves, the cranial nerves develop in a manner similar in some respects to components of the spinal nerves. The somata of motor neuroblasts originate within the neuroepithelium; those of sensory neuroblasts are derived from the neural crest, with additional contributions in the head from ectodermal placodes (Fig. 3.19).
The motor fibres of the cranial nerves that project to striated muscle are the axons of cells originating in the basal plate of the midbrain and hindbrain. The functional and morphological distinction between the neurones within these various nerves is based on the types of muscle innervated. In the trunk, the motor roots of the spinal nerves all emerge from the spinal cord close to the ventral midline, to supply the muscles derived from the somites. In the head, the motor outflow is traditionally divided into two pathways (see Figs. 3.2B, 3.19). General somatic efferent neurones exit ventrally in a similar manner to those of the spinal cord. Thus the oculomotor, trochlear, abducens and hypoglossal nerves parallel the organization of the somatic motor neurones in the spinal cord. The second motor component, the special branchial efferent, consists of the motor parts of the trigeminal, facial, glossopharyngeal and vagus nerves, which supply the pharyngeal (branchial) arches and the accessory nerve. All these nerves have nerve exit points more dorsally placed than in the somatic motor system.
The cranial sensory ganglia are derived in part from the neural crest and in part from cells of the ectodermal placodes (see Figs. 3.13, 3.19). Generally, neurones distal to the brain are derived from placodes, and proximal ones are derived from the neural crest (see Fig. 3.19). Supporting cells of all sensory ganglia arise from the neural crest. The most rostral sensory ganglion, the trigeminal, contains both neural crest– and placode-derived neurones that mediate general somatic afferent functions. The same applies to the more caudal cranial nerves (facial, glossopharyngeal, vagus), but the two cell populations form separate ganglia in the case of each nerve. The proximal series of ganglia is derived from neural crest (forming the proximal ganglion of the facial nerve, the superior ganglion of the glossopharyngeal nerve and the jugular ganglion of the vagus nerve); the distal series is derived from placodal cells (forming the geniculate ganglion of the facial nerve, the petrosal ganglion of the glossopharyngeal nerve and the nodose ganglion of the vagus nerve). These ganglia contain neurones that mediate special, general visceral and somatic afferent functions. The vestibulocochlear nerve has a vestibular ganglion that contains both crest and placodal cells and an acoustic ganglion from placodal neurones only; it conveys special somatic afferents.
Autonomic Nervous System
In the trunk at neurulation, neural crest cells migrate from the neural epithelium to lie transitorily on the fused neural tube. Thereafter, crest cells migrate laterally and then ventrally to their respective destinations (see Fig. 3.12). In the head, the neural crest cells migrate prior to neural fusion, producing a vast mesenchymal population as well as autonomic neurones.
Neurones of the enteric nervous system are described as arising from the vagal crest—that is, the neural crest derived from somite levels 1 to 7, and the sacral crest caudal to the twenty-eighth somite. At all these levels the crest cells also differentiate into glial-like support cells alongside the neurones (Fig. 3.20).
Sympathetic Ganglia
Neural crest cells migrate ventrally within the body segments to penetrate the underlying somites and continue to the region of the future paravertebral and prevertebral plexuses, notably forming the sympathetic chain of ganglia as well as the major ganglia around the ventral visceral branches of the abdominal aorta (see Figs. 3.12, 3.20).
The local environment is the major factor that controls the appropriate differentiation of the presumptive autonomic ganglion neurones. The factors responsible for subsequent adrenergic, cholinergic or peptidergic phenotype have yet to be identified, although it has been proposed that fibronectin and basal lamina components initiate adrenergic phenotypical expression at the expense of melanocyte numbers. Cholinergic characteristics are acquired relatively early, and the appropriate phenotypical expression may be promoted by cholinergic differentiation factor and CNTF.
Enteric Nervous System
The enteric nervous system is derived from the neural crest. The axial levels of crest origin are shown in Figure 3.20. Premigratory neural crest cells are not prepatterned for specific axial levels; rather, they attain their axial value as they leave the neuraxis. Once within the gut wall, there is a regionally specific pattern of enteric ganglia formation that may be controlled by the local splanchnopleuric mesenchyme. Cranial neural crest from somite levels 1 to 7 contributes to the enteric nervous system, forming both neuroblasts and glial support cells.
Central Nervous System
Spinal Cord
The neuroblasts of the lateral walls of the tube are large and initially round or oval (apolar). Soon they develop processes at opposite poles and become bipolar neuroblasts. However, one process is withdrawn, and the neuroblast becomes unipolar, although this is not invariably so in the case of the spinal cord. Further differentiation leads to the development of dendritic processes, and the cells become typical multipolar neurones. In the developing cord they occur in small clusters, representing clones of neurones. Development of a longitudinal sulcus limitans on each side of the central canal of the cord divides the ventricular and intermediate zones in each lateral wall into a basal (ventrolateral) plate or lamina and an alar (dorsolateral) plate or lamina (see Fig. 3.18). This separation indicates a fundamental functional difference. Neural precursors in the basal plate include the motor cells of the anterior (ventral) and lateral grey columns, whereas those of the alar plate exclusively form ‘interneurones’ (which possess both short and long axons), some of which receive the terminals of primary sensory neurones. Caudally the central canal of the cord ends as a fusiform dilatation, the terminal ventricle.
Anterior (Ventral) Grey Column
The cells of the ventricular zone are closely packed at this stage and arranged in radial columns (see Fig. 3.6). Their disposition may be determined in part by contact guidance along the earliest radial array of glial fibres that cross the full thickness of the early neuroepithelium. The cells of the intermediate zone are more loosely packed. They increase in number initially in the region of the basal plate. This enlargement outlines the anterior (ventral) column of the grey matter and causes a ventral projection on each side of the median plane; the floor plate remains at the bottom of the shallow groove produced. As growth proceeds, these enlargements, which are further increased by development of the anterior funiculi (tracts of axons passing to and from the brain), encroach on the groove until it becomes converted into the slit-like anterior median fissure of the adult spinal cord (see Fig. 3.18). The axons of some of the neuroblasts in the anterior grey column cross the marginal zone and emerge as bundles of ventral spinal nerve rootlets on the anterolateral aspect of the spinal cord. These constitute, eventually, both the α-efferents, which establish motor end-plates on extrafusal striated muscle fibres, and the γ-efferents, which innervate the contractile polar regions of the intrafusal muscle fibres of the muscle spindles.
Lateral Grey Column
In the human embryo, the definitive grouping of ventral column cells, which characterizes the mature cord, occurs early; by the fourteenth week (80 mm), all the major groups can be recognized. As the anterior and lateral grey columns assume their final form, the germinal cells in the ventral part of the ventricular zone gradually stop dividing. The layer becomes less thick until it ultimately forms the single-layered ependyma that lines the ventral part of the central canal of the spinal cord.
Posterior (Dorsal) Grey Column
The posterior (dorsal) column develops later; consequently, for a time, the ventricular zone is much thicker in the dorsolateral lamina (alar plate) than it is in the ventrolateral lamina (basal plate) (see Fig. 3.6).
While the columns of grey matter are being defined, the dorsal region of the central canal becomes narrow and slit-like, and its walls come into apposition and fuse with each other (see Fig. 3.18). In this way, the central canal becomes relatively reduced in size and somewhat triangular in outline.
About the end of the fourth week, advancing axonal sprouts invade the marginal zone. The first to develop are those destined to become short intersegmental fibres, derived from neuroblasts in the intermediate zone, and fibres of dorsal roots of spinal nerves that pass into the spinal cord, derived from neuroblasts of the early spinal ganglia. The earlier dorsal root fibres that invade the dorsal marginal zone arise from small dorsal root ganglionic neuroblasts. By the sixth week they form a well-defined oval bundle near the peripheral part of the dorsolateral lamina (see Figs. 3.6, 3.18). This bundle increases in size and, spreading toward the median plane, forms the primitive, fine-calibre posterior funiculus. Later, fibres derived from new populations of large dorsal root ganglionic neuroblasts join the dorsal root; they are destined to become fibres of much larger calibre. As the posterior funiculi increase in thickness, their medial surfaces come into contact, separated only by the posterior medial septum, which is ependymal in origin and neuroglial in nature. It is thought that the displaced primitive posterior funiculus may form the basis of the dorsolateral tract or fasciculus (of Lissauer).
Maturation of the Spinal Cord
The cervical and lumbar enlargements appear at the time their respective limb buds develop.
In early embryonic life, the spinal cord occupies the entire length of the vertebral canal, and the spinal nerves pass at right angles to the cord. After the embryo has attained a length of 30 mm, the vertebral column begins to grow more rapidly than the spinal cord, and the caudal end of the cord gradually becomes more cranial in the vertebral canal (Fig. 3.21). Most of this relative rostral migration occurs during the first half of intrauterine life. By the twenty-fifth week the terminal ventricle of the spinal cord has altered in level from the second coccygeal vertebra to the third lumbar vertebra, a distance of nine segments. Because the change in level begins rostrally, the caudal end of the terminal ventricle, which is adherent to the overlying ectoderm, remains in situ, and the walls of the intermediate part of the ventricle and its covering pia mater become drawn out to form a delicate filament, the filum terminale. The separated portion of the terminal ventricle persists for a time, but it usually disappears before birth. It occasionally gives rise to congenital cysts in the neighbourhood of the coccyx. In the definitive state, the upper cervical spinal nerves retain their position at roughly right angles to the cord. Proceeding caudally, the nerve roots lengthen and become progressively more oblique.
Brain
A summary of the derivatives of the cerebral vesicles from caudal to rostral is given in Table 3.1.
Rhombencephalon
By the time the midbrain flexure appears, the length of the hindbrain is greater than that of the combined extent of the other two brain vesicles. Rostrally it exhibits a constriction, the isthmus rhombencephali (see Fig. 3.2B), which is best viewed from the dorsal aspect. Ventrally the hindbrain is separated from the dorsal wall of the primitive pharynx only by the notochord, the two dorsal aortae and a small amount of mesenchyme; on each side it is closely related to the dorsal ends of the pharyngeal arches.
The pontine flexure appears to ‘stretch’ the thin epithelial roof plate, which becomes widened. The greatest increase in width corresponds to the region of maximal convexity, so the outline of the roof plate becomes rhomboidal. Due to the same change, the lateral walls become separated, particularly dorsally, and the cavity of the hindbrain, subsequently the fourth ventricle, becomes flattened and somewhat triangular in cross-section. The pontine flexure becomes increasingly acute until, at the end of the second month, the laminae of its cranial (metencephalic) and caudal (myelencephalic) slopes are opposed to each other (see Fig. 3.23); at the same time, the lateral angles of the cavity extend to form the lateral recesses of the fourth ventricle.
Cells of the basal plate (ventrolateral lamina)
Cells of the basal plate form three elongated but interrupted columns positioned ventrally and dorsally, with an intermediate column between (Fig. 3.22). The most ventral column is continuous with the anterior grey column of the spinal cord and will supply muscles considered ‘myotomic’ in origin. It is represented in the caudal part of the hindbrain by the hypoglossal nucleus, and it reappears at a higher level as the nuclei of the abducens, trochlear and oculomotor nerves, which are somatic efferent nuclei. The intermediate column is represented in the upper part of the spinal cord and caudal brain stem (medulla oblongata and pons) and is for the supply of branchial (pharyngeal) and postbranchial musculature. It is interrupted and forms the elongated nucleus ambiguus in the caudal brain stem, which gives fibres to the ninth, tenth and eleventh cranial nerves. The latter continues into the cervical spinal cord as the origin of the spinal accessory nerve. At higher levels, parts of this column give origin to the motor nuclei of the facial and trigeminal nerves. These three nuclei are termed branchial (special visceral) efferent nuclei. The most dorsal column of the basal plate (represented in the spinal cord by the lateral grey column) innervates viscera. It too is interrupted, with its large caudal part forming some of the dorsal nucleus of the vagus and its cranial part the salivatory nucleus. These are termed general visceral (general splanchnic) efferent nuclei, and their neurones give rise to preganglionic, parasympathetic nerve fibres.
Cell columns of the alar plate (dorsolateral lamina)
Cell columns of the alar plate are interrupted and give rise to general visceral (general splanchnic) afferent, special visceral (special splanchnic) afferent, general somatic afferent and special somatic afferent nuclei (their relative positions, in simplified transverse section, are shown in Fig. 3.22). The general visceral afferent column is represented by part of the dorsal nucleus of the vagus nerve, the special visceral afferent column by the nucleus of the tractus solitarius, the general somatic afferent column by the afferent nuclei of the trigeminal nerve and the special somatic afferent column by the nuclei of the vestibulocochlear nerve. (The relatively simple functional independence of these afferent columns implied by the foregoing classification is mainly an aid to elementary learning. The emergent neurobiological mechanisms are in fact much more complex and less well understood.) Although they tend to retain their primitive positions, some of these nuclei are later displaced by differential growth patterns, by the appearance and growth of neighbouring fibre tracts and possibly by active migration.
Myelencephalon
The caudal slope of the embryonic hindbrain constitutes the myelencephalon, which develops into the medulla oblongata (see Fig. 3.2). The nuclei of the ninth, tenth, eleventh and twelfth cranial nerves develop in the positions already indicated, and afferent fibres from the ganglia of the ninth and tenth nerves form an oval marginal bundle in the region overlying the alar (dorsolateral) lamina. Throughout the rhombencephalon, the dorsal edge of this lamina is attached to the thin expanded roof plate and is termed the rhombic lip. (The inferior rhombic lip is confined to the myelencephalon; the superior rhombic lip to the metencephalon.) As the walls of the rhombencephalon spread outward, the rhombic lip protrudes as a lateral edge that becomes folded over the adjoining area. The rhombic lip may later become adherent to this area, and its cells migrate actively into the marginal zone of the basal plate. In this way the oval bundle that forms the tractus solitarius becomes buried. Alar plate cells that migrate from the rhombic lip are believed to give rise to the olivary and arcuate nuclei and the scattered grey matter of the nuclei pontis. While this migration is in progress, the floor plate is invaded by fibres that cross the median plane (accompanied by neurones that cluster in and near this plane), and it becomes thickened to form the median raphe. Some of the migrating cells from the rhombic lip in this region do not reach the basal plate and form an oblique ridge: the corpus pontobulbare (nucleus of the circumolivary bundle) across the dorsolateral aspect of the inferior cerebellar peduncle.
Metencephalon
The rostral slope of the embryonic hindbrain is the metencephalon, from which both the cerebellum and the pons develop. Before formation of the pontine flexure, the dorsolateral laminae of the metencephalon are parallel with one another. After its formation, the roof plate of the hindbrain becomes rhomboidal, and the dorsal laminae of the metencephalon lie obliquely. They are close at the cranial end of the fourth ventricle but widely separated at the level of its lateral angles (Fig. 3.23). Accentuation of the flexure approximates the cranial angle of the ventricle to the caudal angle, and the alar plates of the metencephalon now lie almost horizontally.
The basal plate of the metencephalon becomes the pons. Ventricular, intermediate and marginal zones are formed in the usual way, and the nuclei of the trigeminal, abducens and facial nerves develop in the intermediate layer. It is possible that the grey matter of the formatio reticularis is derived from the basal plate and that of the nuclei pontis from the alar plate by the active migration of cells from the rhombic lip. However, at about the fourth month the pons is invaded by corticopontine, corticobulbar and corticospinal fibres; it becomes proportionately thicker and takes on its adult appearance. It is relatively smaller in a full-term neonate.
Fourth ventricle and choroid plexus
Caudal to the developing cerebellum the roof of the fourth ventricle remains epithelial and covers an approximately triangular zone from the lateral angles of the rhomboid fossa to the median obex (see Fig. 3.23). Nervous tissue fails to develop over this region, and vascular pia mater is closely applied to the subjacent ependyma. At each lateral angle and in the midline caudally, the membranes break through, forming the lateral (Luschka) and median (Magendie) apertures of the roof of the fourth ventricle. These become the principal routes by which cerebrospinal fluid, produced in the ventricles, escapes into the subarachnoid space. The vascular pia mater (tela choroidea), in an inverted V formation cranial to the apertures, invaginates the ependyma to form vascular fringes, which become the vertical and horizontal parts of the choroid plexuses of the fourth ventricle.
Cerebellum
The cerebellum develops from the rhombic lip—the dorsal part of the alar plate of the metencephalon, which constitutes the rostral margin of the diamond-shaped fourth ventricle. Two rounded swellings develop that project partly into the ventricle at first (see Fig. 3.23), forming the rudimentary cerebellar hemispheres. The most rostral part of the roof of the metencephalon originally separates the two swellings, but it becomes invaded by cells derived from the alar plate, which form the rudiments of the vermis. At a later stage, extroversion of the cerebellum occurs, its intraventricular projection is reduced and the dorsal extraventricular prominence increases. The cerebellum now consists of a bilobar (dumbbell shaped) swelling stretched across the rostral part of the fourth ventricle (see Fig. 3.23). It is continuous rostrally with the superior medullary velum, formed from the isthmus rhombencephali, and caudally with the epithelial roof of the myelencephalon. With growth, a number of transverse grooves appear on the dorsal aspects of the cerebellar rudiment; these are the precursors of the numerous fissures that characterize the surface of the mature cerebellum (Fig. 3.24).
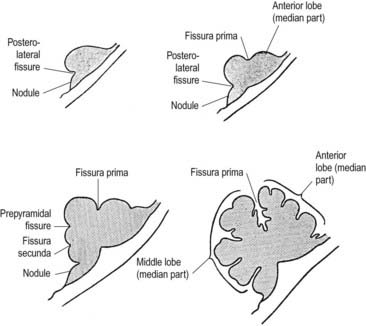
Fig. 3.24 Median sagittal sections through the developing cerebellum, showing four different stages.
The first fissure to appear on the cerebellar surface (see Fig. 3.24) is the lateral part of the posterolateral fissure, which forms the border of a caudal region corresponding to the flocculi of the adult. The right and left parts of this fissure subsequently meet in the midline, where they form the boundary between the most caudal vermian lobule, the nodule and the rest of the vermis. The flocculonodular lobe can now be recognized as the most caudal cerebellar subdivision at this stage, and it serves as the attachment of the epithelial roof of the fourth ventricle. Because of the expansion of the other divisions of the cerebellum, the flocculonodular lobe comes to occupy an anteroinferior position in adults. At the end of the third month a transverse sulcus appears on the rostral slope of the cerebellar rudiment and deepens to form the fissura prima. This cuts into the vermis and both hemispheres and forms the border between the anterior and posterior lobes. Contemporaneously, two short transverse grooves appear in the caudal vermis. The first is the fissura secunda (postpyramidal fissure), which forms the rostral border of the uvula; the second, the prepyramidal fissure, demarcates the pyramid (see Fig. 3.24). The cerebellum now grows dorsally, rostrally, caudally and laterally, and the hemispheres expand much more than does the inferior vermis, which becomes buried at the bottom of a deep hollow, the vallecula. Numerous other transverse grooves develop, the most extensive being the horizontal fissure.
Cellular development of the cerebellum
The cerebellum consists of a cortex beneath which are buried a series of deep nuclei. The organization of the cerebellar cortex is similar to that of the cerebral cortex, except that the latter has six layers and the former has only three. However, whereas in the cerebral cortex neuroblasts originate from the ventricular zone and migrate ventriculofugally toward the pial surface (in an ‘inside-out’ fashion), early in cerebellar development a layer of cells derived exclusively from the metencephalic rhombic lip initially migrates ventriculofugally to form a layer beneath the glia limitans over the surface of the developing cerebellum. These cells form the external germinative layer, and later in development their progeny will migrate ventriculopetally (in an ‘outside-in’ manner) into the cerebellum. Thus, the cerebellum has an intraventricular portion (cells proliferating from the ventricular zone) and an extraventricular portion (cells proliferating from the external germinative layer) during development. The extraventricular portion becomes larger at the expense of the intraventricular part, the so-called extroversion of the cerebellum. Before the end of the third month the main mass of the cerebellum is extraventricular.
The developed cerebellar cortex contains three layers: the molecular layer, the Purkinje layer and the granular layer. The early bilateral expansion of the ventricular surface reflects the production, by the metencephalic alar plate ventricular epithelium, of neuroblasts that will give rise to the radial glia, cerebellar nuclei and efferent neurones of the cerebellar cortex (Purkinje cells) (Fig. 3.25). The radial glia play a role in guiding the Purkinje cells to the meningeal surface of the cerebellar anlage. During this early stage of cerebellar development, which is dominated by the production and migration of efferent cerebellar neurones, the surface of the cerebellar anlage remains smooth. Extroversion of the cerebellum begins later, when cells of the external granular layer, also termed the superficial matrix, begin proliferating and migrating. These cells produce the granule cells, which migrate inward along the radial glia and through the layers of Purkinje cells, settling deep to them in the granular layer. This stage coincides with the emergence of the transverse folial pattern. Proliferation and migration of granule cells lead to a great rostrocaudal expansion of the meningeal surface of the cerebellum, forming the transverse fissures and transforming the multicellular layer of Purkinje cells into a monolayer. Purkinje cells and nuclear cells are formed prior to the granule cells, and granule cells serve as the recipient of the main afferent (mossy fibre) system of the cerebellum. Thus, the development of efferent neurones of the cerebellar cortex and nuclei precedes the development of its afferent organization.
When the external germinative layer is initially formed, the multicellular Purkinje cell layer beneath is not uniform but is subdivided into clusters that form columns extending rostrocaudally (Fig. 3.26). The medial Purkinje cell clusters develop into the future vermis. These Purkinje cells will grow axons that connect to neurones in the vestibular nuclei and the fastigial nucleus. The lateral clusters belong to the future hemispheres and will grow axons terminating in the interposed and dentate nuclei. The sharp border in the efferent projections from the vermis and hemispheres is thus established at an early age. These clusters will give rise to Purkinje cell zones in the adult cerebellum that project to a single vestibular or cerebellar nucleus.
Mesencephalon
The mesencephalon or midbrain is derived from the intermediate primary cerebral vesicle. It persists for a time as a thin-walled tube enclosing a cavity of some size, separated from that of the prosencephalon by a slight constriction and from the rhombencephalon by the isthmus rhombencephali (Figs. 3.2, 3.27). Later, its cavity becomes relatively reduced in diameter, and in the adult brain it forms the cerebral aqueduct. The basal (ventrolateral) plate of the midbrain increases in thickness to form the cerebral peduncles, which are small at first but enlarge rapidly after the fourth month, when their numerous fibre tracts begin to appear in the marginal zone. The neuroblasts of the basal plate give rise to the nuclei of the oculomotor nerve and some grey masses of the tegmentum, while the nucleus of the trochlear nerve remains in the region of the isthmus rhombencephali. The cells giving rise to the trigeminal mesencephalic nucleus arise on either side of the dorsal midline, from the isthmus rhombencephali rostrally across the roof of the mesencephalon. Recent studies have shown that the progenitors of these cells do not express neural crest cell markers.
The cells of the dorsal part of the alar (dorsolateral) plates proliferate and invade the roof plate, which thickens and is later divided into corpora bigemina by a median groove. Caudally this groove becomes a median ridge, which persists in the adult as the frenulum veli. The corpora bigemina are later subdivided into the superior and inferior colliculi by a transverse furrow. The red nucleus, substantia nigra and reticular nuclei of the midbrain tegmentum may first be defined at the end of the third month. Their origins are probably mixed from neuroblasts of both basal and alar plates.
Prosencephalon
At an early stage, a transverse section through the forebrain shows the same parts displayed in similar sections of the spinal cord and medulla oblongata: thick lateral walls connected by thin floor and roof plates. Moreover, each lateral wall is divided into a dorsal area and a ventral area separated internally by the hypothalamic sulcus (see Fig. 3.27). This sulcus ends anteriorly at the medial end of the optic stalk. In the fully developed brain it persists as a slight groove extending from the interventricular foramen to the cerebral aqueduct. It is analogous to, if not the homologue of, the sulcus limitans. The thin roof plate remains epithelial but is invaginated by vascular mesenchyme, the tela choroidea of the choroid plexuses of the third ventricle. Later the lateral margins of the tela undergo a similar invagination into the medial walls of the cerebral hemispheres. The floor plate thickens as the nuclear masses of the hypothalamus and subthalamus develop.
As the most rostral portion of the prosencephalon enlarges, it curves ventrally, and two additional diverticula rapidly expand from it, one on each side. These diverticula are rostrolateral to the optic stalks and subsequently form the cerebral hemispheres. Their cavities are the rudiments of the lateral ventricles, and they communicate with the median part of the forebrain cavity by relatively wide openings that ultimately become the interventricular foramina. The anterior limit of the median part of the forebrain consists of a thin sheet, the lamina terminalis (see Fig. 3.27), which stretches from the interventricular foramina to the recess at the base of the optic stalks. The anterior part of the forebrain, including the rudiments of the cerebral hemispheres, is the telencephalon (endbrain) and the posterior part of the diencephalon (between brain). Both contribute to the formation of the third ventricle, although the latter predominates. The fate of the lamina terminalis is described later.
Diencephalon
The diencephalon is broadly divided by the hypothalamic sulcus into dorsal (pars dorsalis diencephali) and ventral (pars ventralis diencephali) parts; these, however, are composite, and each contributes to diverse neural structures. The dorsal part develops into the (dorsal) thalamus and metathalamus along the immediate suprasulcal area of its lateral wall, while the highest dorsocaudal lateral wall and roof form the epithalamus. The thalamus (see Fig. 3.27) is first visible as a thickening that involves the anterior part of the dorsal area. Caudal to the thalamus, the lateral and medial geniculate bodies, or metathalamus, are first recognizable as surface depressions on the internal aspect and as elevations on the external aspect of the lateral wall. As the thalami enlarge to become smooth ovoid masses, the wide interval between them gradually narrows into a vertically compressed cavity that forms the greater part of the third ventricle. After a time these medial surfaces may come into contact and become adherent over a variable area, the connection (single or multiple) constituting the interthalamic adhesion or massa intermedia. The caudal growth of the thalamus excludes the geniculate bodies from the lateral wall of the third ventricle.
At first the lateral aspect of the developing thalamus is separated from the medial aspect of the cerebral hemisphere by a cleft, but with growth, the cleft becomes obliterated (Fig. 3.28) as the thalamus fuses with the part of the hemisphere in which the corpus striatum is developing. Later, with the development of the projection fibres (corticofugal and corticopetal) of the neocortex, the thalamus becomes related to the internal capsule, which intervenes between it and the lateral part of the corpus striatum (lentiform nucleus). Ventral to the hypothalamic sulcus, the lateral wall of the diencephalon, in addition to median derivatives of its floor plate, forms a large part of the hypothalamus and subthalamus.
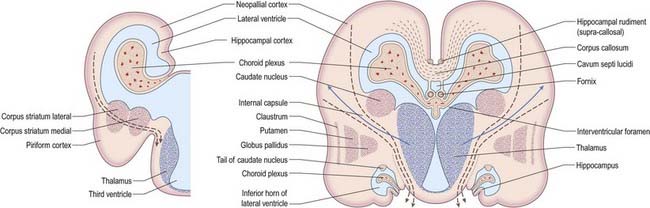
Fig. 3.28 Development of the basal nuclei and internal capsule.
(Redrawn by permission from Hamilton, W.J., Boyd, J.D., Mossman, H.W. 1972. Human Embryology: Prenatal Development of Form and Function. Williams and Wilkins, Baltimore.)
The ventral part of the diencephalon forms the subsulcal lateral walls of the third ventricle and takes part in the formation of the hypothalamus, including the mammillary bodies, the tuber cinereum and the infundibulum of the hypophysis. The mammillary bodies arise as a single thickening, which becomes divided by a median furrow during the third month. Anterior to them, the tuber cinereum develops as a cellular proliferation that extends forward as far as the infundibulum. In front of the tuber cinereum, a wide-mouthed diverticulum forms in the floor of the diencephalon. It grows toward the stomodeal roof and comes into contact with the posterior aspect of a dorsally directed ingrowth from the stomodeum (Rathke’s pouch). These two diverticula together form the hypophysis cerebri (see Fig. 3.15). An extension of the third ventricle persists in the base of the neural outgrowth as the infundibular recess. The remaining caudolateral walls and floor of the ventral diencephalon are an extension of the midbrain tegmentum, the subthalamus. This forms the rostral limits of the red nucleus, substantia nigra, numerous reticular nuclei and a wealth of interweaving, ascending, descending and oblique nerve fibre bundles, which have many origins and destinations.
Third ventricle and choroid plexus
The roof plate of the diencephalon, rostral to the pineal gland (and continuing over the median telencephalon), remains thin and epithelial in character and is subsequently invaginated by the choroid plexuses of the third ventricle (Fig. 3.29). Before the development of the corpus callosum and the fornix, it lies at the bottom of the longitudinal fissure, between and reaching the two cerebral hemispheres. It extends as far rostrally as the interventricular foramina and lamina terminalis. Here and elsewhere, choroid plexuses develop by the close apposition of vascular pia mater and ependyma without intervening nervous tissue. With development, the vascular layer is infolded into the ventricular cavity and develops a series of small villous projections, each covered by a cuboidal epithelium derived from the ependyma. The cuboidal cells carry numerous microvilli on their ventricular surfaces; basally, the plasma membrane becomes complexly folded into the cell. The early choroid plexuses secrete a protein-rich cerebrospinal fluid into the ventricular system, which may provide a nutritive medium for the developing epithelial neural tissues. As the latter becomes increasingly vascularized, the histochemical reactions of the cuboidal cells and the character of the fluid change to the adult type. The remaining lining of the third ventricle does not simply form generalized ependymal cells. Many regions become highly specialized, developing concentrations of tanycytes or other modified cells (e.g. those of the subfornical organ), the organum vasculosum (intercolumnar tubercle) of the lamina terminalis, the subcommissural organ and those lining the pineal, suprapineal and infundibular recesses, which are collectively termed the circumventricular organs.
Telencephalon
The telencephalon (endbrain) consists of two lateral diverticula connected by a median region (the telencephalon impar). The anterior part of the third ventricle develops from the impar and is closed below and in front by the lamina terminalis. The lateral diverticula are outpouchings of the lateral walls of the telencephalon; these may correspond to the alar lamina, although this is uncertain. Their cavities are the future lateral ventricles, and their walls are formed by the presumptive nervous tissue of the cerebral hemispheres. The roof plate of the median part of the telencephalon remains thin and is continuous behind with the roof plate of the diencephalon (see Fig. 3.27). The anterior parts of the hypothalamus, which include the optic chiasma, optic recess and related nuclei, develop in the floor plate and lateral walls of the prosencephalon, ventral to the primitive interventricular foramina. The chiasma is formed by the meeting and partial decussation of the optic nerves in the ventral part of the lamina terminalis. The optic tracts subsequently grow backward from the chiasma to end in the diencephalon and midbrain.
Cerebral hemispheres
The cerebral hemispheres arise as diverticula of the lateral walls of the telencephalon, with which they remain in continuity around the margins of the initially relatively large interventricular foramina, except caudally, where they are continuous with the anterior part of the lateral wall of the diencephalon (see Figs. 3.2, 3.27). As growth proceeds, the hemisphere enlarges forward, upward and backward and acquires an oval outline, medial and superolateral walls and a floor. As a result, the medial surfaces approach, but are separated by, a vascularized mesenchyme and pia mater that fill the median longitudinal fissure (see Fig. 3.29). At this stage the floor of the fissure is the epithelial roof plate of the telencephalon, which is directly continuous caudally with the epithelial roof plate of the diencephalons.
At the early oval stage of hemispheric development, regions are named according to their future principal derivatives. The rostromedial and ventral floor becomes linked with the forming olfactory apparatus and is termed the primitive olfactory lobe. The floor (ventral wall, or base) of the larger remainder of the hemisphere forms the anlage of the primitive corpus striatum and amygdaloid complex, including its associated rim of lateral and medial walls; this is the striate part of the hemisphere. The rest of the hemisphere—the medial, lateral, dorsal and caudal regions—is the suprastriate part of the hemisphere. Although largest in terms of surface area, it initially possesses comparatively thin walls. The rostral end of the oval hemisphere becomes the definitive frontal pole. As the hemisphere expands, its original posterior pole moves relatively in a caudoventral and lateral direction, following a curve like a ram’s horn; it curves toward the orbit in association with the growth of the caudate nucleus (and other structures) to form the definitive temporal pole. A new posterior part persists as the definitive occipital pole of the mature brain (Fig. 3.30). The great expansion of the cerebral hemispheres is characteristic of mammals and especially of humans. In their subsequent growth they overlap, successively, the diencephalon and the mesencephalon and then meet the rostral surface of the cerebellum. The temporal lobes embrace the flanks of the brain stem.
Lateral ventricles and choroid plexus
The early diverticulum or anlage of the cerebral hemisphere initially contains a simple spheroidal lateral ventricle that is continuous with the third ventricle via the interventricular foramen. The rim of the foramen is the site of the original evagination. The expanding ventricle develops the ram’s horn shape of the surrounding hemisphere, becoming first roughly ellipsoid and then a curved cylinder that is convex dorsally (see Fig. 3.30). The ends of the cylinder expand toward, but do not reach, the frontal and (temporary) occipital poles; differentiating and thickening neural tissues separate the ventricular cavities and pial surfaces at all points, except along the line of the choroidal fissure. Pronounced changes in ventricular form accompany the emergence of a temporal pole. The original caudal end of the curved cylinder expands within its substance, and the temporal extensions in each hemisphere pass ventrolaterally to encircle both sides of the upper brain stem. Another extension may develop from the root of the temporal extension in the substance of the definitive occipital pole and pass caudomedially; it is quite variable in size, often asymmetric on the two sides and one or both may be absent. Although the lateral ventricle is a continuous system of cavities, specific parts are now given regional names. The central part (body) extends from the interventricular foramen to the level of the posterior edge (splenium) of the corpus callosum. Three cornua (horns) diverge from the body: anterior toward the frontal pole, posterior toward the occipital pole and inferior toward the temporal pole.
The pia mater covering the epithelial roof of the third ventricle at this stage is itself covered with loosely arranged mesenchyme and developing blood vessels. These vessels subsequently invaginate the roof of the third ventricle on each side of the median plane to form its choroid plexuses. The lower part of the medial wall of the cerebral hemisphere, which immediately adjoins the epithelial roof of the interventricular foramen and the anterior extremity of the diencephalon, also remains epithelial. It consists of ependyma and pia mater; elsewhere the walls of the hemispheres are thickening to form the pallium. The thin part of the medial wall of the hemisphere is invaginated by vascular tissue that is continuous in front with the choroid plexus of the third ventricle and constitutes the choroid plexus of the lateral ventricle. This invagination occurs along a line that arches upward and backward, parallel with and initially limited to the anterior and upper boundaries of the interventricular foramen. This curved indentation of the ventricular wall, where no nervous tissue develops between ependyma and pia mater, is termed the choroidal fissure (see Figs. 3.27C, 3.28). Subsequent assumption of the definitive form of the choroidal fissure depends on related growth patterns in neighbouring structures. Of particular importance are the relatively slow growth of the interventricular foramen, the secondary ‘fusion’ between the lateral diencephalon and medial hemisphere walls, the encompassing of the upper brain stem by the forward growth of the temporal lobe and its pole toward the apex of the orbit and the massive expansion of two great cerebral commissures (the fornix and corpus callosum). The choroidal fissure is now clearly a caudal extension of the much reduced interventricular foramen, which arches above the thalamus and here is only a few millimetres from the median plane. Near the caudal end of the thalamus it diverges ventrolaterally, its curve reaching and continuing in the medial wall of the temporal lobe over much of its length (i.e. to the tip of the inferior horn of the lateral ventricle). The upper part of the arch will be overhung by the corpus callosum, and throughout its convexity it is bordered by the fornix and its derivatives.
Basal nuclei
At first, growth proceeds more actively in the floor and the adjoining part of the lateral wall of the developing hemisphere, and elevations formed by the rudimentary corpus striatum encroach on the cavity of the lateral ventricle (see Figs. 3.27, 3.28). The head of the caudate nucleus appears as three successive parts—medial, lateral and intermediate—which produce elevations in the floor of the lateral ventricle. Caudally these merge to form the tail of the caudate nucleus and the amygdaloid complex, both of which remain close to the temporal pole of the hemisphere. When the occipital pole grows backward and the general enlargement of the hemisphere carries the temporal pole downward and forward, the tail of the caudate is continued from the floor of the central part (body) of the ventricle into the roof of its temporal extension, the future inferior horn. The amygdaloid complex encapsulates its tip. Anteriorly the head of the caudate nucleus extends forward to the floor of the interventricular foramen, where it is separated from the developing anterior end of the thalamus by a groove; later the head expands in the floor of the anterior horn of the lateral ventricle. The lentiform nucleus develops from two laminae of cells, medial and lateral, which are continuous with both the medial and lateral parts of the caudate nucleus. The internal capsule appears first in the medial lamina and extends laterally through the outer lamina to the cortex. It divides the laminae in two; the internal parts join the caudate nucleus, and the external parts form the lentiform nucleus. In the latter, the remaining medial lamina cells give rise mainly to the globus pallidus and the lateral lamina cells to the putamen. The putamen subsequently expands concurrently with the intermediate part of the caudate nucleus.
Fusion of diencephalic and telencephalic walls
As the hemisphere enlarges, the caudal part of its medial surface overlaps and hides the lateral surface of the diencephalon (thalamic part), from which it is separated by a narrow cleft occupied by vascular connective tissue. At this stage (about the end of the second month) a transverse section made caudal to the interventricular foramen would pass from the third ventricular cavity successively through the developing thalamus, the narrow cleft just mentioned, the thin medial wall of the hemisphere and the cavity of the lateral ventricle, with the corpus striatum in its floor and lateral wall (see Fig. 3.28).
As the thalamus increases in extent it acquires a superior surface in addition to medial and lateral surfaces. The lateral part of its superior surface fuses with the thin medial wall of the hemisphere so that this part of the thalamus is finally covered with the ependyma of the lateral ventricle immediately ventral to the choroidal fissure. As a result, the corpus striatum is approximated to the thalamus and is separated from it only by a deep groove that becomes obliterated by increased growth along the line of contact. The lateral aspect of the thalamus is now in continuity with the medial aspect of the corpus striatum, so that a secondary union between the diencephalon and telencephalon is effected over a wide area, providing a route for the subsequent passage of projection fibres to and from the cortex (see Fig. 3.28).
Formation of the insula
At the end of the third month, while the corpus striatum is developing, there is a relative restriction of growth between the frontal and temporal lobes. The region lateral to the striatum becomes depressed to form a lateral cerebral fossa, with a portion of cortex, the insula, at its base (see Fig. 3.30). As the temporal lobe continues to protrude toward the orbit, and with more rapid growth of the temporal and frontal cortices, the surface of the hemisphere expands at a rate greater than that of the hemisphere as a whole, and the cortical areas become folded, forming gyri and sulci. The insula is gradually overgrown by these adjacent cortical regions, and they overlap it to form the opercula, the free margins of which form the anterior part of the lateral fissure. This process is not completed until after birth. The lentiform nucleus remains deep to and coextensive with the insula.
Olfactory nerve, limbic lobe and hippocampus
The growth changes in the temporal lobe that help submerge the insula produce important changes in the olfactory and neighbouring limbic areas. As it approaches the hemispheric floor, the olfactory tract diverges into lateral, medial and (variable) intermediate striae. The medial stria is clothed with a thin archaeocortical medial olfactory gyrus. This curves up into other archaeocortical areas anterior to the lamina terminalis (paraterminal gyrus, prehippocampal rudiment, parolfactory gyrus, septal nuclei), and these continue into the indusium griseum. The lateral stria, clothed by the lateral olfactory gyrus, and, when present, the intermediate stria terminate in the rostral parts of the piriform area. This includes the olfactory trigone and tubercle, anterior perforated substance and uncus (hook) and entorhinal area of the anterior part of the future parahippocampal gyrus. Its lateral limit is indicated by the rhinal sulcus. The forward growth of the temporal pole and the general expansion of the neocortex cause the lateral olfactory gyrus to bend laterally, with the summit of the convexity lying at the anteroinferior corner of the developing insula (Fig. 3.31). During the fourth and fifth months, much of the piriform area becomes submerged by the adjoining neocortex, and in the adult, only part of it remains visible on the inferior aspect of the cerebrum.
The limbic (bordering) lobe is the first part of the cortex to differentiate. At first it forms a continuous, almost circular strip on the medial and inferior aspects of the hemisphere. Below and in front, where the stalk of the olfactory tract is attached, it constitutes part of the piriform area. The portion outside the curve of the choroid fissure (Fig. 3.32) constitutes the hippocampal formation. In this region the neural progenitors of the developing cortex proliferate and migrate, and the wall of the hemisphere thickens and produces an elevation that projects into the medial side of the ventricle: this elevation is the hippocampus. It appears first on the medial wall of the hemisphere in the area above and in front of the lamina terminalis (paraterminal area) and gradually extends backward, curving into the region of the temporal pole, where it adjoins the piriform area. The marginal zone in the neighbourhood of the hippocampus is invaded by neuroblasts to form the dentate gyrus. Both extend from the paraterminal area backward, above the choroid fissure, and follow its curve downward and forward toward the temporal pole, where they continue into the piriform area. A shallow groove (the hippocampal sulcus) crosses the medial surface of the hemisphere throughout the hippocampal formation.
Projection fibres and internal capsule
The growth of the neocortex and its enormous expansion during the latter part of the third month are associated with the initial appearance of corticofugal and corticopetal projection fibres and the pathway they follow, the internal capsule. These fibres follow the route provided by the apposition of the lateral aspect of the thalamus with the medial aspect of the corpus striatum, and as they do so, they divide the latter almost completely into a lateral part, the lentiform nucleus, and a medial part, the caudate nucleus; these two nuclei remain confluent only in their anteroinferior regions (see Figs. 3.28, 3.30). The corticospinal tracts begin to develop in the ninth week of fetal life and have reached their caudal limits by the twenty-ninth week. The fibres destined for the cervical and upper thoracic regions and involved in innervation of the upper limbs are in advance of those concerned with the lower limbs, which in turn are in advance of those concerned with the face. The appearance of reflexes in these three parts of the body follows a comparable sequence.
The majority of subcortical nuclear masses receive terminals from descending fibres of cortical origin. These are joined by thalamocortical, hypothalamocortical and other afferent ascending bundles. The internal capsular fibres pass lateral to the head and body of the caudate nucleus, anterior cornu and central part of the lateral ventricle, rostroventral extensions and body of the fornix and dorsal thalamus and dorsal choroidal fissure; they pass medial to the lentiform nucleus (see Fig. 3.28).
Formation of gyri and sulci
Apart from the shallow hippocampal sulcus and the lateral cerebral fossa, the surfaces of the hemisphere remain smooth and uninterrupted until early in the fourth month (see Fig. 3.31). The parieto-occipital sulcus appears at about that time on the medial aspect of the hemisphere. Its appearance seems to be associated with an increase in the number of splenial fibres in the corpus callosum. Over the same period, the posterior part of the calcarine sulcus appears as a shallow groove extending forward from a region near the occipital pole. It is a true infolding of the cortex in the long axis of the striate area and produces an elevation, the calcar avis, on the medial wall of the posterior horn of the ventricle.
During the fifth month the cingulate sulcus appears on the medial aspect of the hemisphere, and sulci appear on the inferior and superolateral aspects in the sixth month. The central, precentral and postcentral sulci appear, each in upper and lower parts; these two parts usually coalesce shortly afterward, although they may remain discontinuous. The superior and inferior frontal, intraparietal, occipital, superior and inferior temporal, occipitotemporal, collateral and rhinal sulci all make their appearance during the same period. By the end of the eighth month all the important sulci can be recognized (see Fig. 3.31).
Development of commissures
The development of the commissures causes a profound alteration in the medial wall of the hemisphere. At the time of their appearance, the two hemispheres are connected to each other by the median part of the telencephalon. The roof plate of this area remains epithelial, while its floor becomes invaded by the decussating fibres of the optic nerves and developing hypothalamic nuclei. These two routes are thus not available for the passage of commissural fibres from hemisphere to hemisphere across the median plane; these fibres therefore pass through the anterior wall of the interventricular foramen, the lamina terminalis. The first commissures to develop are those associated with the palaeocortex and archicortex. Fibres of the olfactory tracts cross in the ventral or lower part of the lamina terminalis and, together with fibres from the piriform and prepiriform areas and the amygdaloid bodies, form the anterior part of the anterior commissure (Figs. 3.32, 3.33). In addition, the two hippocampi become interconnected by transverse fibres that cross from fornix to fornix in the upper part of the lamina terminalis as the commissure of the fornix. Various other decussating fibre bundles (known as the supraoptic commissures, although they are not true commissures) develop in the lamina terminalis immediately dorsal to the optic chiasma, between it and the anterior commissure.
The commissures of the neocortex develop later and follow the pathways already established by the commissures of the limbic system. Fibres from the tentorial surface of the hemisphere join the anterior commissure and constitute its larger posterior part. All the other commissural fibres of the neocortex associate themselves closely with the commissure of the fornix and lie on its dorsal surface. These fibres increase enormously in number, and the bundle rapidly outgrows its neighbours to form the corpus callosum (see Figs. 3.32, 3.33).
The corpus callosum originates as a thick mass connecting the two cerebral hemispheres around and above the anterior commissure. (This site has been called the precommissural area, but this term has been rejected here because of increasing use of the adjective ‘precommissural’ to denote the position of parts of the limbic lobe—prehippocampal rudiment, septal areas and nuclei and strands of the fornix—in relation to the anterior commissure of the mature brain.) The upper end of this neocortical commissural area extends backward to form the trunk of the corpus callosum. The rostrum of the corpus callosum develops later and separates part of the rostral end of the limbic area from the remainder of the cerebral hemisphere. Further backward growth of the trunk of the corpus callosum then results in the entrapped part of the limbic area becoming stretched out to form the bilateral septum pellucidum. As the corpus callosum grows backward, it extends above the choroidal fissure, carrying the commissure of the fornix on its undersurface. In this way a new floor is formed for the longitudinal fissure, and additional structures come to lie above the epithelial roof of the third ventricle. In its backward growth, the corpus callosum invades the area hitherto occupied by the upper part of the archaeocortical hippocampal formation, and the corresponding parts of the dentate gyrus and hippocampus are reduced to vestiges, the indusium griseum and the longitudinal striae (see Figs. 3.32, 3.33). However, the posteroinferior (temporal) archaeocortical regions of both the dentate gyrus and the hippocampus persist and enlarge.
Cellular Development of the Cerebrum
The earliest migration of neuronal precursors from the ventricular and intermediate zones occurs radially until they approach, but do not reach, the pial surface. Their somata become arranged as a transient cortical plate. Subsequently, proliferation wanes in the ventricular zone but persists for considerable periods in the immediately subjacent subventricular zone. From the pial surface inward, the following zones may be defined: marginal, cortical plate, subplate, intermediate, subventricular and ventricular (see Fig. 3.5). The marginal zone gives rise to the outermost layer of the cerebral cortex, and the neuroblasts of the cortical plate and subplate form the neurones of the remaining cortical laminae (the complexity varies in different locations and with further additions of neurones from the deeper zones). The intermediate zone gradually transforms into the white matter of the hemisphere. Meanwhile, other deep progenitor cells produce generations of glioblasts that also migrate into the more superficial layers. As proliferation wanes and finally ceases in the ventricular and subventricular zones, their remaining cells differentiate into general or specialized ependymal cells, tanycytes or subependymal glial cells.
Mechanisms of cortical development
Rakic (2003) initially demonstrated the migration of neuroblasts along radial glial processes, and this has subsequently been seen to occur in three phases. First, the neuroblasts become apposed to the radial glial cells and establish an axis of polarity away from the ventricular surface. Next, they are propelled along the glial surface until they ‘recognize’ their final destination, whereupon they cease locomotion and detach from the glial processes. They then continue to differentiate according to their final position, and later-born neuroblasts migrate past them toward the pial surface (see Fig. 3.5, Figs. 3.34, 3.35). Cortical neurones or cerebellar granule cells appear equally capable of migrating on hippocampal or cerebellar Bergmann glia, indicating conservation of migration mechanisms in different brain regions.
In a plane perpendicular to its laminae (i.e. tangentially-circumferentially), the cortex is divided into a number of areas, displaying a hierarchy of organization. These include primary areas such as the motor cortex, unimodal association areas concerned with the integration of information from one of the primary areas and multimodal association areas that integrate information from more than one modality. There are also areas concerned with functions that are even less well understood, such as the frontal lobes, concerned with goal-orientation responsibility and long-term planning. The primary areas are further divided into somatotopic maps. At the finest level, the cortex is known to consist of a series of ‘columns’ 50 to 500 µm wide, within which cells on a vertical traverse display common features of modality and electrophysiological responses to stimuli (e.g. the ocular dominance columns of the visual cortex). Despite the precise stacking of neurones in these columns, only 80% to 85% of cells migrate radially along the glial cells; a subpopulation is thought to move tangentially in the intermediate zone (O’Rourke et al 1995). Moreover, some neurones may migrate tangentially on the radial glial cells, as a result of glial cell branching in the cortical plate. The ventricular zone is not the only source of cortical neurones, because striatal and GABAergic neurones are known to migrate from the lateral ganglionic eminence into the developing cortex.
Two models have been proposed to explain the development of this complex cortical organization. The ‘protocortex’ model assumes that the proliferative ventricular epithelium is a ‘tabula rasa’ that generates homogeneous layers of neurones that are patterned solely by the ingrowth of processes from the thalamus. The ‘protomap’ hypothesis proposes that the intrinsic differences between the various areas are specified prior to cell migration (Rakic 1988, 2003). The radial glial cells translate this map from the ventricular zone to the cortical plate, where the pattern is refined by innervating axons. In this ‘radial unit’ model, the tangential coordinates of the different areas are determined by the position of their ventricular ancestors, whereas their radial position is determined by their time of birth and rate of migration.
But what would constitute such a protomap? The investigation of gene expression patterns shows that the early cortex is not homogeneous and that it expresses some markers that are transient and some that persist into adulthood. For example, the mouse gene Id2 marks the transition between the motor and somatosensory cortices in the embryo, whereas limbic-associated membrane protein (LAMP) delineates the limbic cortex throughout life. LAMP expression is regulated by transforming growth factor-α, which is expressed by the lateral ganglionic eminence at the lateral edge of the cortex; the medial edge or cortical hem expresses signalling molecules of the WNT and BMP families. Any or all of these may be the components of short-range signalling centres along the edges of the cortex. Coupled with the gradients of transcription factors such as Emx2 and Lhx2, there is evidence to support the protomap hypothesis (Donoghue and Rakic 1999).
However, studies of cell migration are consistent with the idea that cortical areas might not be rigidly determined. Manipulations of the developing cortex by deafferentation or manipulation of inputs give some indication of the state of commitment of cortical areas. In two independent sets of experiments, somatosensory or auditory cortex was induced to process visual information by misrouting retinal axons to somatosensory thalamus or auditory thalamus (von Melchner, Pallas and Sur, 2000). When the lateral geniculate nucleus and the visual cortex were ablated and space was created in the medial geniculate by ablating the inferior colliculus, cells in the somatosensory or auditory cortex were visually driven, and receptive field and response properties resembled those seen in the visual cortex. These results suggest that the modality of a sensory thalamic nucleus or cortical area can be specified by inputs during development.
How are region-specific projections generated? Layer 5 neurones in various cortical areas extend axons to different repertoires of targets. For instance, layer 5 neurones of the visual cortex project to the tectum, pons and mesencephalic nuclei, whereas those in the motor cortex project to mesencephalic and pontine targets, the inferior olive and dorsal column nuclei and the spinal cord. An interesting feature of these cortical projections is that they arise by collateral formation rather than by projection of the primary axon or by growth cone bifurcation. In the case of the corticopontine projection, collaterals are elicited by a diffusible, chemotrophic agent. Retrograde labelling of neurones at various times in development has shown that rather than being generated de novo, these patterns seem to arise by the pruning of collaterals from a more widespread projection. Visual cortical neurones possess a projection to the spinal cord early in development, which is later eliminated. This late emergence of the specificity of projections could be driven by intrinsic programming of the neurones to be pruned or by a response to position-dependent factors. There is evidence that the latter is the case. When pieces of visual cortex were transplanted into motor areas and the resulting layer 5 projections were labeled later in development, projections to the spinal cord persisted rather than being eliminated, as in normal development. Thus, position plays an important role in the modelling of cortical projections, implying that the same classes of neurones exist in different tangential regions of the cortex. Regressive events such as axon and synapse elimination and neuronal death thus play an important part in modelling the cortex. For example, in rodents, approximately 30% of cortical neurones die, and the number of cells in layer 4 is governed by thalamic input.
Neonatal Brain and Reflexes
The sulci of the cerebral hemispheres appear from the fourth month of gestation (see Fig. 3.31), and at full term the general arrangement of sulci and gyri is present, but the insula is not completely covered. The central sulcus is situated farther rostrally and the lateral sulcus is more oblique than in the adult. Most of the developmental stages of sulci and gyri have been identified in the brains of premature infants. Of the cranial nerves, the olfactory and the optic at the chiasma are much larger than in the adult, whereas the roots of the other nerves are relatively smaller.
Reflexes present at birth
A number of reflexes are present at birth, and their demonstration is used to indicate normal development of the nervous system and responding muscles. Five tests of neurological development are most useful in determining gestational age. The pupillary reflex is consistently absent before 29 weeks’ gestation and present after 31 weeks; the glabellar tap, a blink in response to a tap on the glabella, is absent before 32 weeks and present after 34 weeks; the neck righting reflex appears between 34 and 37 weeks; the traction response, in which flexion of the neck or arms occurs when the baby is pulled up by the wrists from the supine position, appears after 33 weeks; head turning in response to light appears between 32 and 36 weeks. The spinal reflex arc is fully developed by the eighth week of gestation, and lower limb flexor tone is detectable from about 29 weeks. The extensor plantar (Babinski’s) response, which involves extension of the great toe with spreading of the remaining toes in response to stimulation of the lateral aspect of the sole of the foot, is elicited frequently in neonates; it reflects poor cortical control of motor function by the immature brain. Generally, reflexes develop as muscles gain tone. They appear in a sequential manner from caudal to cephalic, in the lower limb before the upper, and centripetally, with distal reflexes appearing before proximal ones (Allen and Capute 1990).
Difficulties in sucking and swallowing in infancy may be an early indication of disturbed nervous system function. There is an interesting correlation between the feeding styles of neonates and later eating habits. Children who were obese at 1 and 2 years of age, as measured by triceps skinfold thickness, had a feeding pattern in the first month of life that was characterized by sucking more rapidly, producing higher pressures during prolonged bursts of sucking and having shorter periods between bursts of sucking. Fewer feeds and higher sucking pressure seem to be associated with greater adiposity.
Meninges
During development the meninges can be divided into the pachymeninx (dura mater) and the leptomeninges (arachnoid mater, subarachnoid space with arachnoid cells and fibres and pia mater). All meningeal layers are derived from loose mesenchyme that surrounds the developing neural tube, termed the meninx primitiva or primary meninx. (For a detailed account of development of the meninges in the human, consult O’Rahilly and Muller 1986.)
Growth of the cranial vault is initiated from ossification centres within the desmocranial mesenchyme. A wave of osteodifferentiation moves radially outward from these centres, stopping when adjacent bones meet at regions where sutures are induced to form. Once sutures are formed, a second phase of development occurs in which growth of the cranial bones occurs at the sutural margins. This growth forms most of the skull. A number of hypotheses have been generated to explain the process of suture morphogenesis. It has been suggested that the dura mater contains fibre tracts that extend from fixed positions in the cranial base to sites of dural reflection underlying each of the cranial sutures, and the tensional forces so generated dictate the position of the sutures and locally inhibit precocious ossification. Other hypotheses support the concept of local factors in the calvaria that regulate suture morphogenesis. Following removal of the entire calvaria, the skull regenerates and sutures and bones develop in anatomically correct positions, suggesting that the dura can dictate suture position at least in regeneration of the neonatal calvaria. In transplants of sutures in which the fetal dura mater was left intact, a continuous fibrous suture remained between developing vault bones, whereas in transplants in which the fetal dura mater was removed, bony fusion occurred (Opperman et al 1993).
Cranial Arteries (Fig 3.36)
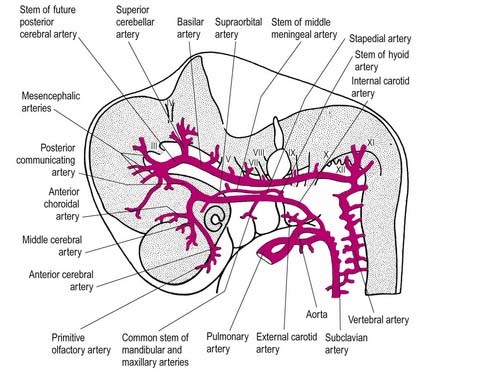
Fig. 3.36 Origins of the main cranial arteries.
(After Padget, D.H. 1948. The development of cranial arteries in the human embryo. Contrib. Embryol. Carnegie Inst. Washington 32, 205–261, by permission.)
Meningeal Arteries
At stages 20 to 23 (7 to 8 weeks’ gestation), further expansion of the cerebral hemispheres completes the circle of Willis, with development of the anterior communicating arteries by 8 weeks’ gestation. An anular network of meningeal arteries originates, mainly from each middle cerebral artery, and passes over each developing cerebral hemisphere. Caudally, similar meningeal branches arise from the vertebral and basilar arteries and embrace the cerebellum and brain stem. The further development of the telencephalon somewhat obscures this early pattern over the cerebrum.
Vascularization of the Brain
The meningeal perforating branches pass into the brain parenchyma as cortical, medullary and striate branches (Fig. 3.37). The cortical vessels supply the cortex via short branches that may form precapillary anastomoses, whereas the medullary branches supply the white matter. The latter converge toward the ventricle but rarely reach it; they often follow a tortuous course as they pass around bundles of nerves. The striate branches, which penetrate the brain through the anterior perforated substance, supply the basal nuclei and internal capsule via a sinuous course; they are larger than the medullary branches, and the longest ones reach close to the ventricle. The periventricular region and basal nuclei are also supplied by branches from the tela choroidea, which develops from the early pial plexus but becomes medially and deeply placed as the telencephalon enlarges.
The cortical and medullary branches irrigate a series of corticosubcortical cone-shaped areas, centred around a sulcus containing an artery. They supply a peripheral portion of the cerebrum and are grouped as ventriculopetal arteries. Striate branches, in contrast, arborize close to the ventricle and supply a more central portion of the cerebrum; together with branches from the tela choroidea, they give rise to ventriculofugal arteries. The latter supply the ventricular zone (germinal matrix of the brain) and send branches toward the cortex. The ventriculopetal and ventriculofugal arteries run toward each other but do not make any connections or anastomoses (see Fig. 3.37); however, the ventriculopetal arteries form networks of small arterioles. The ventriculopetal vessels supply relatively more mature regions of the brain compared with the ventriculofugal vessels, which are subject to constant remodelling and do not develop tunicae mediae until ventricular zone proliferation is complete. The boundary zone between these two systems (outer centripetal and inner centrifugal) has practical implications related to the location of ischaemic lesions (periventricular leukomalacia [PVL]) in the white matter of premature infants’ brains. Although it was thought that the distribution of ischaemic lesions in PVL coincided with the demarcation zone between the centrifugal and centripetal vascular arterial systems, this is no longer thought to provide the complete answer. Three major interacting factors contribute to the pathology seen in PVL: the incomplete state of development of the vasculature in the ventricular zone, the maturation-dependent impairment of cerebral bloodflow regulation in premature infants and the vulnerability of oligodendroblasts in the periventricular region, which are particularly affected by swings in cerebral ischaemia and reperfusion (Volpe 2001).
By 32 to 34 weeks, marked involution of the ventricular zone (germinal matrix) has occurred, and the cortex acquires its complex gyral pattern and an increased vascular supply. Ventricular zone capillaries are gradually remodelled to blend with the capillaries of the caudate nucleus. Heubner’s artery eventually supplies only a small area at the medial aspect of the head of the caudate nucleus. In the cortex there is progressive elaboration of cortical blood vessels (see Fig. 3.37), and toward the end of the third trimester, the balance of cerebral circulation shifts from one that is central and basal nuclei oriented to one that predominantly serves the cortex and white matter. These changes in the pattern of cerebral circulation are of major significance in the pathogenesis and distribution of hypoxic-ischaemic lesions in the developing human brain. In a premature brain the majority of ischaemic lesions occur in the boundary zone between the centripetal and centrifugal arteries, that is, in the periventricular white matter. In the full-term infant the cortical boundary zones and watershed areas between different arterial blood supplies are similar to those in adults.
Vessels of the ventricular zone (germinal matrix)
Vessel density is relatively low in the ventricular zone, suggesting that this area may normally have a relatively low bloodflow. Immature vessels, without a complex basal lamina or glial sheet, have been described up to 26 weeks’ gestation in the zone; the endothelium of these vessels is apparently thinner than in the cortical vessels. In infants of less than 30 weeks’ gestation, the vessels in the ventricular zone contain no smooth muscle, collagen or elastic fibres. Collagen and smooth muscle are seen in other regions after 30 weeks’ gestation but are not detected in the remains of the germinal matrix. The lack of these components could make the vessels in this zone vulnerable to changes in intraluminal pressure, and the lack of smooth muscle would preclude them from participating in autoregulatory processes. Cerebral vessels in premature infants lack elastic fibres and have a disproportionately small number of reticulin fibres. Comparison of the cortical and germinal plate blood vessels shows that in infants between 25 and 32 weeks’ gestation, the germinal matrix vessels commonly consist of one or two endothelial cells with an occasional pericyte, and the capillary lumina are larger than those of the vessels in the cortex. In more mature infants, the basal lamina is thicker and more irregular when compared with cortical vessels.
Veins of the Head
The earliest vessels form a transitory primordial hindbrain channel that drains into the precardinal vein (Fig. 3.38). This is soon replaced by the primary head vein, which runs caudally from the medial side of the trigeminal ganglion, lateral to the facial and vestibulocochlear nerves and otocyst, then medial to the vagus nerve, to become continuous with the precardinal vein. A lateral anastomosis subsequently brings it lateral to the vagus nerve. The cranial part of the precardinal vein forms the internal jugular vein.
The primary capillary plexus of the head becomes separated into three fairly distinct strata by the differentiation of the skull and meninges. The superficial vessels, draining the skin and underlying soft parts, eventually discharge in large part into the external jugular system. They retain some connections with the deeper veins through so-called emissary veins. Deep to this is the venous plexus of the dura mater, from which the dural venous sinuses differentiate. This plexus converges on each side into anterior, middle and posterior dural stems (see Fig. 3.37). The anterior stem drains the prosencephalon and mesencephalon and enters the primary head vein rostral to the trigeminal ganglion. The middle stem drains the metencephalon and empties into the primary head vein caudal to the trigeminal ganglion, while the posterior stem drains the myelencephalon into the start of the precardinal vein. The deepest capillary stratum is the pial plexus, from which the veins of the brain differentiate. It drains at the dorsolateral aspect of the neural tube into the adjacent dural venous plexus. The primary head vein also receives, at its cranial end, the primitive maxillary vein, which drains the maxillary prominence and region of the optic vesicle.
The vessels of the dural plexus undergo profound changes, largely to accommodate the growth of the cartilaginous otic capsule of the membranous labyrinth and expansion of the cerebral hemispheres. With growth of the otic capsule, the primary head vein is gradually reduced, and a new channel joining the anterior, middle and posterior dural stems appears dorsal to the cranial nerve ganglia and the capsule. Where this new vessel joins the middle and posterior stems, together with the posterior dural stem itself (see Fig. 3.37B), the adult sigmoid sinus is formed.
Development of the Eye
Development of the eye involves a series of interactions between neighbouring tissues in the head. These are the neuroectoderm of the forebrain, which forms the sensory retina and accessory pigmented structures; the surface ectoderm, which forms the lens and cornea; and the intervening neural crest mesenchyme, which contributes to the fibrous coats of the eye. These interactions lead to the potential to form optic vesicles throughout a broad anterior domain of neuroectoderm. Subsequent interactions between mesenchyme and neuroectoderm subdivide this region into bilateral domains at the future sites of the eyes. The parallel process of lens determination appears to depend on a brief period of inductive influence that spreads through the surface ectoderm from the rostral neural plate and elicits a lens-forming area of the head. Reciprocal interactions necessary for the complete development of both tissues take place as the optic vesicle forms and contacts the potential lens ectoderm (Saha et al 1992). Vascular tissue of the developing eye may form by local angiogenesis or vasculogenesis of angiogenetic mesenchyme. (Accounts of eye development are given in O’Rahilly 1966 and 1983.)
Embryonic Components of the Eye
The first morphological sign of eye development is a thickening of the diencephalic neural folds at 22 days’ postovulation, when the embryo has seven or eight somites. This optic primordium extends on both sides of the neural plate and crosses the midline at the primordium chiasmatis. A slight transverse indentation, the optic sulcus, appears in the inner surface of the optic primordium on each side of the brain. During the period when the rostral neuropore closes, at about 24 days, the walls of the forebrain at the optic sulcus begin to evaginate, projecting laterally toward the surface ectoderm so that, by 25 days, the optic vesicles are formed. The lumen of each vesicle is continuous with that of the forebrain. Cells delaminate from the walls of the optic vesicle and, probably joined by head mesenchyme and cells derived from the mesencephalic neural crest, invest the vesicle in a sheath of mesenchyme. By 28 days, regional differentiation is apparent in each of the source tissues of the eye. The optic vesicle is visibly differentiated into its three primary parts. Thus, a thick-walled region marks the future optic stalk at the junction with the diencephalon; laterally, the tissue that will become the sensory retina forms a flat disc of thickened epithelium in close contact with the surface ectoderm; and the thin-walled part of the vesicle that lies between these regions will later form the pigmented layer of the retina. The area of surface ectoderm that is closely apposed to the optic vesicle also thickens to form the lens placode. The mesenchymal sheath of the vesicle begins to show signs of angiogenesis. Between 32 and 33 days’ postovulation, the lens placode and optic vesicle undergo coordinated morphogenesis. The lens placode invaginates, forming a pit that pinches off from the surface ectoderm to form the lens vesicle. The surface ectoderm re-forms a continuous layer that will become the corneal epithelium. The lateral part of the optic vesicle also invaginates to form a cup, the inner layer of which—facing the lens vesicle—will become the sensory retina, and the outer layer of which will become the pigmented retinal epithelium. As a result of these folding movements, what were the apical (luminal) surfaces of the two layers of the cup now face each other across a much reduced lumen, the intraretinal space. The pigmented layer becomes attached to the mesenchymal sheath, but the junction between the pigmented and sensory layers is less firm and is the site of pathological detachment of the retina. The two layers are continuous at the lip of the cup, which, at the end of the third month, grows around the front of the lens and forms the pigmented iris. Between the base of the cup and the brain, the narrow part of the optic vesicle forms the optic stalk. The anteroventral surface of the vesicle and distal part of the stalk are also infolded, forming a wide groove—the choroid fissure—through which mesenchyme extends with the associated hyaloid artery. As growth proceeds, the fissure closes, and the artery is included in the distal part of the stalk. Failure of the optic fissure to close is a rare anomaly that is always accompanied by a corresponding deficiency in the choroid and iris (congenital coloboma).
Differentiation of the Functional Components of the Eye
The optic cup becomes patterned, from the base to the rim, into regions with distinct functions (Fig. 3.39). The external stratum remains as a rather thin layer of cells that begin to acquire pigmented melanosomes and form the pigmented epithelium of the retina at around 36 days. In a parallel process that began before invagination, the cells of the inner layer of the cup proliferate to form a thick epithelium. The inner layer forms neural tissue over the base and sides of the cup and non-neural tissue around the lip. The non-neural epithelium is further differentiated into the components of the prospective iris at the rim and the ciliary body a little farther back adjacent to the neural area. The development of this pattern is reflected in regional differences in the expression of various genes that encode transcriptional regulators and are therefore likely to play key roles in controlling and coordinating development. Each of these genes is expressed prior to overt cell type differentiation. For example, PAX6 is expressed in the prospective ciliary and iris regions of the optic cup. Individuals heterozygous for mutations in PAX6 lack an iris, which suggests a causal role for this gene in the development of the iris. The genes expressed in the eye are also active at a variety of other specific sites in the embryo, and this may account, in part, for the co-involvement of the eye and other organs in syndromes that result from single genetic lesions.
Optic Nerve
The optic nerve develops from the optic stalk. The centre of the optic cup, where the optic fissure is deepest, later forms the optic disc. Here the neural retina is continuous with the corresponding invaginated cell layer of the optic stalk; consequently, the developing nerve fibres of the ganglion cells pass directly into the wall of the stalk and convert it into the optic nerve. The fibres of the optic nerve begin to acquire their myelin sheaths shortly before birth, but the process is not completed until sometime later. The optic chiasma is formed by the meeting and partial decussation of the fibres of the two optic nerves in the ventral part of the lamina terminalis at the junction of the telencephalon and the diencephalon in the floor of the third ventricle. Beyond the chiasma, the fibres are continued backward as the optic tracts and pass principally to the lateral geniculate bodies and to the superior tectum.
Lens
The lens develops from the lens vesicle (see Fig. 3.39A). Initially, this is a ball of actively proliferating epithelium that encloses a clump of disintegrating cells; by 37 days, there is a discernible difference between the thin anterior (outward-facing) epithelium and the thickened posterior epithelium. Cells of the posterior wall lengthen and fill the vesicle (see Fig. 3.39B, C) and reduce the original cavity to a slit by about 44 days. The posterior cells become filled with a very high concentration of proteins (crystallins), which renders them transparent. They also become densely packed within the lens as primary lens fibres. Cells at the equatorial region of the lens elongate and contribute secondary lens fibres to the body of the lens in a process that continues into adult life, sustained by continued proliferation of cells in the anterior epithelium. The polarity and growth of the lens appear to depend on the differential distribution of soluble factors that promote either cell division or lens fibre differentiation and are present in the anterior chamber and vitreous humour, respectively.
Differentiation of Structures around the Eye
Extraocular Muscles
The extrinsic ocular muscles derive from prechordal mesenchyme that ingresses at the primitive node very early in development. The prechordal cells lie at the rostral tip of the notochordal process and remain mesenchymal after the notochordal process becomes epithelial and gains a basal lamina. The prechordal mesenchyme migrates laterally toward the paraxial mesenchyme. Although this is a singular origin for muscle, the early myogenic properties of these cells have been demonstrated experimentally; moreover, if transplanted into limb buds, the cells are able to develop into muscle tissue (Wachtler and Jacob 1986).
Eyelids
The eyelids are formed as small cutaneous folds of surface ectoderm and neural crest mesenchyme (see Fig. 3.39C). During the middle of the third month, their edges come together and unite over the cornea to enclose the conjunctival sac, and they usually remain united until about the end of the sixth month. When the eyelids open, the conjunctivae lining their inner surfaces and covering the white (scleral) region of the eye fuse with the corneal epithelium. The eyelashes and the lining cells of the tarsal (meibomian), ciliary and other glands that open onto the margins of the eyelids are all derived from the tarsal plate. Orbicularis oculi develops from skeletal myoblasts that invade the eyelids from the second pharyngeal arch. Levator palpebrae superioris develops from the prechordal mesenchyme and is attached to the upper eyelids by tendons derived from the neural crest. Smooth muscle also develops within the eyelids.
Lacrimal Apparatus
The epithelium of the alveoli and the ducts of the lacrimal gland arise as a series of tubular buds from the ectoderm of the superior conjunctival fornix. These buds are arranged in two groups: one forms the gland proper, and the other forms its palpebral process (de la Cuadra-Blanco, Peces-Peňa and Mèrida-Velasco 2003). The lacrimal sac and nasolacrimal duct are derived from ectoderm in the nasomaxillary groove between the lateral nasal process and the maxillary process of the developing face. This thickens to form a solid cord of cells, the nasolacrimal ridge, which sinks into the mesenchyme. During the third month, the cord becomes canalized to form the nasolacrimal duct. The lacrimal canaliculi arise as buds from the cranial extremity of the cord, which establish openings (puncta lacrimalia) on the margins of the lids. The inferior canaliculus isolates a small part of the lower eyelid to form the lacrimal caruncle and plica semilunaris.
Development of the Ear
Inner Ear
The rudiments of the internal ears appear shortly after those of the eyes as two patches of thickened surface epithelium—the otic placodes—lateral to the hindbrain. The early otic epithelium, which is derived from the otic placode, initiates and then suppresses chondrogenesis in the surrounding periotic mesenchyme. Sonic hedgehog protein, FGFs, and transforming growth factor-β have all been shown to be active in the early stages of otic capsule development in the mouse (Frenz et al 1994).
Each otic placode invaginates as an otic pit while also giving cells to the statoacoustic (vestibulocochlear) ganglion (see Fig. 3.2). The mouth of the pit then closes to form an otocyst (auditory or otic vesicle) (Fig. 3.40). The otocyst is initially piriform, but a vertical infolding of its wall progressively marks off a tubular diverticulum on the medial side, which differentiates into the ductus and saccus endolymphaticus. The latter both communicate via the ductus with the remainder of the vesicle, the utriculosaccular chamber, which is placed laterally. Three compressed diverticula emerge as disc-like evaginations from the dorsal part of this chamber. The central parts of their walls coalesce and disappear, and their peripheral portions persist as the semicircular ducts. The anterior duct is completed first, and the lateral last. A medially directed evagination arises from the ventral part of the utriculosaccular chamber and coils progressively as the cochlear duct. Its proximal extremity becomes constricted and forms the ductus reuniens.
External Ear
Development of the auricle is initiated by the appearance of six hillocks that form around the margins of the dorsal portion of the hyomandibular groove at the 4-mm stage. Three of the six are on the caudal edge of the mandibular arch, and three are on the cranial edge of the hyoid arch. These hillocks appear at stage 15; they tend to be less obvious before that stage. Of those on the mandibular arch, only the most ventral, which subsequently forms the tragus, can be identified at earlier stages. The rest of the auricle is formed in the mesenchyme of the hyoid arch, which extends forward around the dorsal end of the remnants of the hyomandibular groove, forming a keel-like elevation that is the forerunner of the helix. The mandibular arch’s contribution to the auricle is greatest at the end of the second month, and it becomes relatively reduced as growth continues; eventually, the area of skin supplied by the mandibular nerve extends little above the tragus. The lobule is the last part of the auricle to develop.
Allen, Capute, 1990Allen M.C. Capute A.J. Tone and reflex development before term. J. Pediatr. 85:1990;393-399.
Provides details of the development of reflexes in extremely premature infants.
Barkovich A.J., Kuzniedky R.I., Jackson G.D., Guerrini R., Dobyns W.B. A developmental and genetic classification for malformations of cortical development. Neurology. 2005;65:1873-1887.
Begbie, Graham, 2001Begbie J. Graham A. The ectodermal placodes: a dysfunctional family. Phil. Trans. R Soc. Lond. B. Biol. Sci. 356:2001;1655-1660.
Brown, Keynes, Lumsden, 2001Brown M. Keynes R. Lumsden A. The Developing Brain. 2001. Oxford. Oxford University Press.
Covers the main mechanisms of neural development from neurulation to synaptic reorganization.
de la Cuadra-Blanco C., Peces-Peňa M.D., Mèrida-Velasco J.R. Morphogenesis of the human lacrimal gland. J. Anat. 2003;203:531-536.
Donoghue, Rakic, 1999Donoghue M.J. Rakic P. Molecular gradients and compartments in the embryonic primate cerebral cortex. Cereb. Cortex. 9:1999;586-600.
Frenz D.A., Liu W., Williams J.D., Hatcher V., Galinovic-Schwartz V., Flanders K.C., Van de Water T.R. Induction of chondrogenesis: requirement for synergistic interaction of basic fibroblast growth factor and transforming growth factor-beta. Development. 1994;120:415-424.
Gardner, W.J., 1973. The dysraphic states from syringomyelia to anencephaly. Excerpta Medica Amsterdam
Gordon-Weeks P.R. Neuronal Growth Cones. Cambridge University Press: Cambridge; 2000.
Krumlauf, Marshall, Studer, Nonchev, Sham, Lumsden, 1993Krumlauf R. Marshall H. Studer M. Nonchev S. Sham M.H. Lumsden A. Hox homeobox genes and regionalisation of the nervous system. J. Neurobiol. 24:1993;1328-1340.
Le Douarin, Teillet, Catala, 1998Le Douarin N. Teillet M. Catala M. Neurulation in amniote vertebrates: a novel view deduced from the use of quail–chick chimeras. Int. J. Dev. Biol. 42:1998;909-916.
Explores the mechanisms that contribute to secondary neurulation using chimeric techniques.
Muller F., O’Rahilly R. The timing and sequence of appearance of neuromeres and their derivatives in staged human embryos. Acta. Anat. (Basel). 1997;158:83-99.
Opperman, Sweeney, Redmon, Persing, Ogle, 1993Opperman L.A. Sweeney T.M. Redmon J. Persing J.A. Ogle R.C. Tissue interactions with underlying dura mater inhibit osseous obliteration of developing cranial sutures. Dev. Dynam. 198:1993;312-322.
Examines the role of the dura mater in the development of the skull bones and sutures.
O’Rahilly R. The early development of the eye in staged human embryos. Contrib. Embryol. Carnegie. Inst. 1966;38:1.
O’Rahilly R. The timing and sequence of events in the development of the human eye and ear during the embryonic period proper. Anat. Embryol. 1983;168:87-99.
O’Rahilly R., Muller F. The meninges in human development. J. Neuropathol. Exp. Neurol. 1986;45:588-608.
O’Rourke N.A., Sullivan D.P., Kaznowski C.E., Jacobs A.A., McConnell S.K. Tangential migration of neurons in the developing cerebral cortex. Development. 1995;121:2165-2176.
Rakic, 1988Rakic P. Specification of cerebral cortical areas. Science. 241:1988;170-176.
Rakic, 2003Rakic P. Developmental and evolutionary adaptations of cortical radial glia. Cereb. Cortex. 13:2003;541-549.
Saha, Servetnick, Grainger, 1992Saha M.S. Servetnick M. Grainger R.M. Vertebrate eye development. Curr. Opin. Genet. Dev. 2:1992;582-588.
Reviews the interactions involved and genes responsible for eye development.
Volpe J.J. Neurology of the Newborn., Unit I, Human Brain Development, fifth ed. Saunders; 2008.
Volpe J.J. Neurobiology of periventricular leukomalacia in the premature infant. Pediatr. Res. 2001;50:553-562.
von, Melchner, Pallas, Sur, 2000von, Melchner L. Pallas S.L. Sur M. Visual behaviour mediated by retinal projections directed to the auditory pathway. Nature. 404:2000;820-821.
Wachtler F., Jacob M. Origin and development of the cranial skeletal muscles. Bibl. Anat. 1986;29:24-46.
Withington, Beddington, Cooke, 2001Withington S. Beddington R. Cooke J. Foregut endoderm is required at head process stage for anteriormost neural patterning in chick. Development. 128:2001;309-320.