CHAPTER 24 Development of the nervous system
The entire nervous system and the special sense organs originate from three sources, each derived from specific cell populations of the early epiblast termed neural ectoderm. The first source to be clearly delineated is the neural plate, which gives rise to the central nervous system, the somatic motor nerves and the preganglionic autonomic nerves. The second source is from cells at the perimeter of the neural plate, neural crest cells, which remove themselves by epithelial/mesenchymal transition from the plate and migrate away just prior to its fusion into a neural tube. These cells give rise to the majority of the neurones and glia of the peripheral nervous system, i.e. the somatic sensory nerves, the somatic and autonomic ganglia, postganglionic autonomic nerves and suprarenal and chromaffin cells. They also give rise to significant mesenchymal populations in the head. The third source is from ectodermal placodes, which are focal thickening of the ectoderm covering the embryonic head. They contribute cells to the cranial sensory neurones, and form the olfactory epithelia, the epithelia of the inner ear and, by a non-neuronal contribution, the lens of the eye.
NEURULATION
Primary neurulation begins at stage 9 and is completed during stage 12 (Fig. 24.1). The process, although continuous spatially and temporally, has been envisaged as four stages. It begins with local elongation of the ectoderm cells in a midline zone of the embryonic disc and their reorganization into a pseudostratified epithelium, the neural plate. This is followed by reshaping and bending of the neural plate into a neural groove which subsequently closes to form into a neural tube bidirectionally from the midportion to its cranial and caudal ends. A continuous surface ectoderm forms dorsal to the tube.
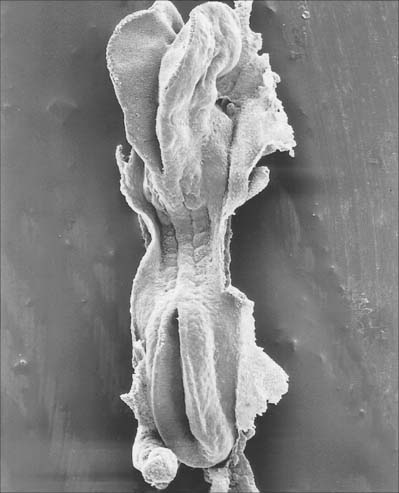
(Photograph by P Collins; printed by S Cox, Electron Microscopy Unit, Southampton General Hospital.)
Fusion of the neural tube starts in embryos with 4–6 somites, at the level of somites 1 and 2, forming the future rhombencephalon. The tube closes caudally and rostrally, forming sequentially cervical and thoracic cord regions, then mesencephalic and prosencephalic brain regions. Rostrally two sites of fusion can be seen. The initial fusion termed α, or the dorsal lip of the rostral neuropore, proceeds caudorostrally. A second site, termed β, or the terminal lip of the rostral neuropore, closes from the rostral end of the neural plate and proceeds rostrocaudally (O’Rahilly & Müller 2002). Closure of these lips of the rostral neuropore is completed when 19–20 pairs of somites are present. Caudal neuropore closure starts when approximately 29 somites are present and the site of closure corresponds to the level of the future somite 31 (the level of the future second sacral vertebra) (O’Rahilly & Müller 2004).
EARLY BRAIN REGIONS
Prior to the closure of the neural tube, the neural folds become considerably expanded in the head region; the first indication of a brain and its major divisions can be seen at stage 10 (Fig. 24.2). Regional expansions, prosencephalon (forebrain), mesencephalon (midbrain), and rhombencephalon (hindbrain), have been called the three primary cerebral vesicles, although the term ‘vesicle’ in this context has been considered inappropriate to describe localized accelerations of growth in the wall of the brain. As the neural tube closes, the neural wall appears to form a series of ridges and depressions perpendicular to its long axis. These transient repeating segments are termed primary neuromeres, and initially six can be identified: the prosencephalon, mesencephalon and four subdivisions of the rhombencephalon, rhombomeres A, B, C and D. The primary neuromeres themselves become subdivided during stages 10, 11 and 12 and a total of 16 secondary neuromeres have been identified. The prosencephalon gives rise to the telencephalon, diencephalon 1 (D1) and diencephalon 2 (D2), which has three subdivisions. The mesencephalon is subdivided into mesencephalon 1 (M1) and mesencephalon 2 (M2). The rhombencephalon is subdivided into the isthmus rhombencephali and rhombomeres 1–8; the original rhombomere A gives rise to secondary rhombomeres 1, 2 and 3; rhombomere B gives rise to secondary rhombomere 4; rhombomere C gives rise to secondary rhombomeres 5, 6 and 7; and rhombomere D gives rise to secondary rhombomere 8 (O’Rahilly & Müller 1999). As the rhombencephalon grows the rhombomeric boundaries become less distinct. With the early appearance of the cerebellum from the isthmus rhombencephali and rhombomere 1, the rhombocephalon is traditionally divided into the metencephalon, which extends to about rhombomere 3, and the myelencephalon, which extends to the spinomedullary junction. A summary of the derivative of the cerebral regions is given in Table 24.1 on page 376.
Table 24.1 Derivatives of the cerebral regions from caudal to rostral
Rhombencephalon (or hindbrain) | |
1. Myelencephalon |
The elongation of the brain occurs at the same time as the appearance of three flexures, which also appear prior to the closure of the neural tube; two are concave ventrally and one concave dorsally. During stages 13 and 14 the brain bends at the mesencephalon (mesencephalic flexure) so that the prosencephalon bends in a ventral direction around the cephalic end of the notochord and foregut until its floor lies almost parallel with that of the rhombencephalon (Fig. 24.3). A bend also appears at the junction of the rhombencephalon and spinal cord (cervical flexure). This increases from the fifth to the end of the seventh week, by which time the rhombencephalon forms nearly a right angle to the spinal cord. However, after the seventh week, extension of the head takes place and the cervical flexure diminishes and eventually disappears. The third bend, the pontine flexure, is directed ventrally between the metencephalon and myelencephalon. It does not substantially affect the outline of the head. In this region, the roof plate thins until it is composed only of a single layer of cells and pia mater, the tela choroidea. The flexure of the neural tube at this point produces a rhombic shape in the roof which later forms the medullary velum.
EARLY CELLULAR ARRANGEMENT OF THE NEURAL TUBE
Histologically the early neural tube is composed of a pseudostratified neuroepithelium. It extends from the inner aspect of the tube to the outer limiting basal lamina and the surrounding pia mater. The epithelium contains stem cells which will give rise to populations of neuroblasts and glioblasts. A population of radial glia differentiates very early and provides a scaffold for later cells to follow. As development proceeds, three zones or layers develop (Figs 24.4–24.6). These are an internal ventricular zone (variously termed the germinal, primitive ependymal or matrix layer), in which mitosis occurs, and which contains the nucleated parts of the columnar cells and rounded cells undergoing mitosis; a middle, mantle zone (also termed the intermediate zone) which contains the migrant cells from the divisions occurring in the ventricular zone; and an outer, marginal zone, which initially consists of the external cytoplasmic processes of the radial glia. The latter is soon invaded by tracts of axonal processes which grow from neuroblasts developing in the mantle zone, together with varieties of non-neuronal cells (glial cells and later vascular endothelium and perivascular mesenchyme). For further development of these layers see pages 368–369.
At first the neural tube caudal to the brain is oval in transverse section and its lumen is narrow and slit-like (Fig. 24.4). The original floor plate and the dorsal site of fusion of the tube initially contain non-neural cells. With cellular proliferation, the lateral walls thicken and the lumen, now the central canal, widens in its dorsal part and is somewhat diamond-shaped on cross-section (Fig. 24.6). The widening of the canal is associated with the development of a longitudinal sulcus limitans on each side which divides the ventricular and mantle (intermediate) zones in each lateral wall into a ventrolateral lamina or basal plate and a dorsolateral lamina or alar plate respectively. This separation underlies a fundamental functional difference.
Throughout the neural tube there is a generic pattern in the position of the neurones which is specified by the juxtaposition of the notochord to the neural tube. Experimental lateral or dorsal grafting of a notochord results in the induction of a floor plate overlying the grafted notochord and the induction of ectopic motor neurones dorsally. Similarly, lateral or dorsal grafts of a floor plate also result in the induction of a new floor plate overlying the graft and the induction of ectopic dorsal motor neurones. Removal of the notochord results in the elimination of the floor plate and the motor neurones and the differentiation of dorsal cell types in the ventral region of the cord (Fig. 24.7).
FAILURE OF NEURULATION
Failure of neurulation produces the conditions of craniorachischisis totalis (where the entire neural tube is unfused in the dorsal midline), cranioschisis or anencephaly (where the neural tube is fused dorsally to form the spinal cord but is not fused dorsally in the brain), and spina bifida (where local regions of the spinal neural tube are unfused, or there is failure of formation of the vertebral neural arches). (Fig. 24.8; see also Ch. 44). Anencephalic fetuses display severe disturbances in the shape, position and ossification of the basichondrocranium and in the course of the intracranial notochord and so the condition is more complicated than ‘simply’ a failure of neural tube fusion.
NEURAL CREST
The neuronal populations of the early epiblast become arranged in the medial region of the embryonic disc as the neural plate. Laterally, neural folds or crests indicate the transitional region between neural and surface ectoderm. Along most of the neuraxis the cells at the tips of the neural folds undergo an epithelial/mesenchyme transformation. They acquire migratory properties and leave the epithelium just prior to its fusion with the contralateral fold in the dorsal midline. The migratory cells so formed are collectively termed the neural crest. Cells within the rostral prosencephalic neural fold and smaller populations of cells in bilateral sites lateral to the early brain do not form migratory neural crest cells but remain within the surface epithelium as ectodermal placodes.
Neural crest populations arise from the neural folds as primary neurulation proceeds and simultaneously progresses rostrally and caudally. Crest cells migrate from the neural folds of the brain prior to tube closure. Caudally, from approximately somite 29, secondary neurulation processes produce the most caudal neural crest. Two distinct populations of neural crest cells are formed: a neuronal population produced throughout the brain and spinal cord which gives rise to sensory and autonomic neurones and glia, and a non-neuronal mesenchymal population which arises only from the brain (Figs 24.9 and 24.10). Melanocytes develop from a subpopulation of neural crest cells derived from both the head and trunk. They form one of the three pigment cell types (the others being retinal pigment epithelium and the pigment cells of the pineal organ, which both originate from the diencephalon).
In the trunk the migration patterns of neural crest cells is channelled by the somites. As the crest cells move laterally and ventrally they can pass between the somites and within the rostral sclerotomal half of each somite, but they cannot penetrate the caudal moiety of the sclerotomal mesenchyme. Thus the segmental distribution of the spinal and sympathetic ganglia is imposed on the neural crest cells by a prepattern that exists within the somitic paraxial mesenchyme (Fig. 24.11). The origin of the cranial-caudal patterning of the ventral neural crest cells is not clear.
Rostral to the otic vesicle, neural crest cells arise from specific regions of the brain. Early in development, a number of transverse subdivisions perpendicular to the long axis of the brain can be seen within the rhombencephalon, dividing it into segments termed rhombomeres (Müller & O’Rahilly 1997). Eight main rhombomeres extend from the midbrain–hindbrain boundary rostrally to the spinal cord caudally (Fig. 24.2). Rhombomeres 8 and 7 give rise to neural crest cells which migrate into the fourth and sixth pharyngeal arches; rhombomere 6 crest cells invade pharyngeal arch three. Rhombomere 4 crest cells migrate into arch 2, whereas rhombomeres 5 and 3 give rise to a very small number of neural crest cells which migrate rostrally and caudally to enter the adjacent even-numbered neighbours. Rhombomeres 1 and 2 produce crest cells which invade the first pharyngeal arch. In each rhombomere, mesenchymal populations and the sensory and autonomic ganglia are formed from the crest cells (see Fig. 12.4).
ECTODERMAL PLACODES
Prior to neural tube closure, the elevating neural folds contain two distinctive neuronal populations. The larger population of neural crest cells migrates from the neural epithelium prior to neural tube fusion. A smaller population of neuroepithelial cells becomes incorporated into the surface ectoderm after neural tube closure. These areas of neuroepithelium within the surface ectoderm have been termed ectodermal placodes. Although the majority of the ectodermal placodes form nervous tissue, non-neurogenic placodes also occur (Begbie & Graham 2001). After an appropriate inductive stimulus, the placodes thicken and either generate migratory neuronal cells that will contribute to the cranial sensory ganglia, or the whole placodal region invaginates to form a vesicle beneath the remaining surface ectoderm. Neurogenic placodes undergo both processes. Paired non-neurogenic placodes invaginate to form the lens vesicles under the inductive influence of the optic vesicles (see Ch. 41).
The neural folds meet in the rostral midline adjacent to the buccopharyngeal membrane. This rostral neural fold does not generate neural crest but gives rise to the hypophysial placode, i.e. the future Rathke’s pouch, which remains within the surface ectoderm directly rostral to the buccopharyngeal membrane. The rostral neural fold also gives rise to the olfactory placodes, which remain as paired, laterally placed placodes, and to the epithelium of the nasal cavity (Fig. 24.10).
Further caudally, similar neurogenic placodes can be identified and divided into three categories, namely the epibranchial, otic and trigeminal placodes (Fig. 24.12). The epibranchial placodes appear in the surface ectoderm immediately dorsal to the area of pharyngeal (branchial) cleft formation. The first epibranchial placode is located at the level of the first pharyngeal groove and contributes cells to the distal (geniculate) ganglion of the facial nerve, the second and third epibranchial placodes contribute cells to the distal ganglia of the glossopharyngeal (petrosal) and vagus (nodose) nerves respectively. These placodes thicken and cells begin to detach from their epithelium soon after the pharyngeal pouches have contacted the overlying ectoderm. Concurrently the neural crest cells reach and move beyond these lateral extensions of the pharynx. Neurones migrate from the epibranchial placodes internally to the sites of ganglion formation, where they show signs of early differentiation into neurones, including the formation of neurites.
The otic placodes, located lateral to the myelencephalon, invaginate to form otic vesicles from which the membranous labyrinth of the ear develops. Neurones of the vestibulocochlear nerve ganglia arise from neurones that bud off the ventromedial aspect of the otic cup, after which they can be distinguished in the acoustic and vestibular ganglia (see Ch. 38).
PITUITARY GLAND (HYPOPHYSIS CEREBRI)
The most rostral portion of the neural plate, which will form the hypothalamus, is in contact rostrally with the future adenohypophysis in the rostral neural ridge, and caudally with the neurohypophysis, in the floor of the neural plate (Fig. 24.10). After neurulation the cells of the rostral neural ridge remain in the surface ectoderm and form the hypophysial placode which is in close apposition and adherent to the overlying prosencephalon.
Neural crest mesenchyme later moves between the prosencephalon and surface ectoderm except at the region of the placode. Before rupture of the buccopharyngeal membrane, proliferation of the periplacodal mesenchyme means that the placode forms the roof and walls of a saccular depression. This hypophysial recess (pouch of Rathke, Figs 24.13 and 24.14) is the rudiment of the adenohypophysis. It lies immediately ventral to the dorsal border of the buccopharyngeal membrane, extending in front of the rostral tip of the notochord, and retaining contact with the ventral surface of the prosencephalon. It is constricted by continued proliferation of the surrounding mesenchyme to form a closed vesicle, but remains for a time connected to the ectoderm of the stomodeum by a solid cord of cells, which can be traced down the posterior edge of the nasal septum. Masses of epithelial cells form mainly on each side and in the ventral wall of the vesicle, and the development of the adenohypophysis progresses by the ingrowth of a mesenchymal stroma. Differentiation of epithelial cells into stem cells and three differentiating types is said to be apparent during the early months of fetal development. It has been suggested that different types of cells arise in succession, and that they may be derived in differing proportions from different parts of the hypophysial recess. A craniopharyngeal canal, which sometimes runs from the rostral part of the hypophysial fossa of the sphenoid to the exterior of the skull, is often said to mark the original position of the hypophysial recess. Traces of the stomodeal end of the recess are usually present at the junction of the septum of the nose with the palate. Others have claimed that the craniopharyngeal canal itself is a secondary formation caused by the growth of blood vessels, and is quite unconnected with the stalk of the adenohypophysis.
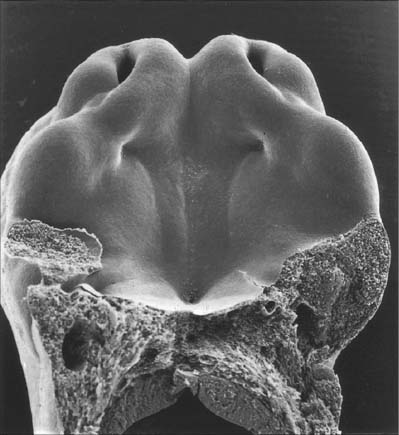
(Photograph by P Collins; printed by S Cox, Electron Microscopy Unit, Southampton General Hospital.)
Just caudal to, but in contact with, the adenohypophysial recess, a hollow diverticulum elongates towards the stomodeum from the floor of the neural plate just caudal to the hypothalamus (Fig. 24.14B); this region of neural outgrowth is the neurohypophysis. It forms an infundibular sac, the walls of which increase in thickness until the contained cavity is obliterated except at its upper end, where it persists as the infundibular recess of the third ventricle. The neurohypophysis becomes invested by the adenohypophysis, which extends dorsally on each side of it. The adenohypophysis gives off two processes from its ventral wall which grow along the infundibulum and fuse to surround it, coming into relation with the tuber cinereum and forming the tuberal portion of the hypophysis. The original cavity of Rathke’s pouch remains first as a cleft, and later as scattered vesicles, and can be identified readily in sagittal sections through the mature gland. The dorsal wall of Rathke’s pouch remains thin and fuses with the adjoining part of the neurohypophysis as the pars intermedia.
NEUROGLIA
The ventricular zone lining the early central canal of the spinal cord and the cavities of the brain gives rise to neurones and glial cells (Figs 24.4 and 24.5). One specialized form of glial cell is the radial glial cell, whose radial processes extend both outwards to form the outer limiting membrane deep to the pia mater, and inwards, to form the inner limiting membrane around the central cavity. The geometry of these cells may provide contact guidance paths for cell migrations, both neuronic and glioblastic. A secondary radial glial scaffold is formed in the late developing cerebellum and dentate gyrus and serves to translocate neurones, formed in secondary germinal centres, to their definitive adult locations. Radial glia eventually lose their connections with both inner and outer limiting membranes, except those persisting in the retina as Müller cells, in the cerebellum as Bergmann glia and in the hypothalamus as tanycytes. They can differentiate into neurones as well as astrocytes. They may partially clothe the somata of neighbouring developing neurones (between presumptive synaptic contacts), or similarly enwrap the intersynaptic surfaces of their neurites. Glial processes may expand around intraneural capillaries as perivascular end-feet. Other glioblasts retain an attachment (or form new expansions) to the pia mater, the innermost stratum of the meninges, as pial end-feet. Glioblasts also line the central canal and cavities of the brain as generalized or specialized ependymal cells, but lose their peripheral attachments. In some situations, as in the anterior median fissure of the spinal cord, ependymal cells retain their attachments to both the inner and outer limiting membranes. Thus, glia function as perineuronal satellites, and provide cellular channels interconnecting extracerebral and intraventricular cerebrospinal fluid, the cerebral vascular bed, the intercellular crevices of the neuropil and the cytoplasm of all neural cell varieties.
MECHANISMS OF NEURAL DEVELOPMENT
HISTOGENESIS OF THE NEURAL TUBE
The wall of the early neural tube consists of an internal ventricular zone (sometimes termed the germinal matrix) abutting the central lumen. It contains the nucleated parts of the pseudostratified columnar neuroepithelial cells and rounded cells undergoing mitosis. The early ventricular zone also contains a population of radial glial cells whose processes pass from the ventricular surface to the pial surface, thus forming the internal and external glia limitans (glial limiting membrane). As development proceeds the early pseudostratified epithelium proliferates and an outer layer, the marginal zone, devoid of nuclei but containing the external cytoplasmic processes of cells, is delineated. Subsequently a middle, mantle layer (intermediate zone) forms as the newly formed neurones migrate ventriculofugally from the ventricular zone (see Fig. 24.5).
Most CNS cells are produced in the proliferative zone adjacent to the future ventricular system, and in some regions this area is the only actively mitotic zone. According to the monophyletic theory of neurogenesis it is assumed to produce all cell types. The early neural epithelium, including the deeply placed ventricular mitotic zone, consists of a homogeneous population of pluripotent cells whose varying appearances reflect different phases in a proliferative cycle. The ventricular zone is considered to be populated by a single basic type of progenitor cell and to exhibit three phases. The cells show an ‘elevator movement’ as they pass through a complete mitotic cycle, progressively approaching and then receding from the internal limiting membrane (Fig. 24.15). DNA replication occurs while the cells are extended and their nuclei approach the pial surface; they then enter a premitotic resting period while the cells shorten and their nuclei pass back towards the ventricular surface. The cells now become rounded close to the internal limiting membrane and undergo mitosis. They then elongate and their nuclei move towards the outer edge during the postmitotic resting period, after which DNA synthesis commences once more and the cycle is repeated. The cells so formed may then either start another proliferative cycle or migrate outwards (i.e. radially) and differentiate into neurones as they approach and enter the adjacent stratum. This differentiation may be initiated as they pass outwards during the postmitotic resting period. The proliferative cycle continues with the production of clones of neurones and glioblasts. This sequence of events has been called inter-kinetic nuclear migration: it eventually declines. At the last division two postmitotic daughter cells are produced and they differentiate at the ventricular surface into ependyma.
The progeny of some of these divisions move away from the ventricular zone to form an intermediate zone of neurones. The early spinal cord and much of the brain stem shows only these three main layers, i.e. ventricular, intermediate and marginal zones. However, in the telencephalon the region of cellular proliferation extends deeper than the ventricular zone where the escalator movement of interkinetic migration is seen, and a subventricular zone appears between the ventricular and intermediate layers (Fig. 24.15). Here cells continue to multiply to provide further generations of neurones and glia which subsequently migrate into the intermediate and marginal zones. In some regions of the nervous system (e.g. the cerebellar cortex) some mitotic subventricular stem cells migrate across the entire neural wall to form a subpial population, and establish a new zone of cell division and differentiation. Many cells formed in this site remain subpial in position, but others migrate back towards the ventricle through the developing nervous tissue, and finish their migrations in various definitive sites where they differentiate into neurones or macroglial cells. In the cerebral hemispheres, a zone termed the cortical plate is formed outside the intermediate zone by radially migrating cells from the ventricular zone. The most recently formed cells migrate to the outermost layers of the cortical plate, so that earlier formed and migrating cells become subjacent to those migrating later. In the forebrain there is an additional transient stratum deep to the early cortical plate, the subplate zone.
LINEAGE AND GROWTH IN THE NERVOUS SYSTEM
Growth cones
During development, the growing axons of neuroblasts navigate with precision over considerable distances, often pursuing complex courses to reach their targets. Eventually they make functional contact with their appropriate end organs (neuromuscular endings, secreto-motor terminals, sensory corpuscles or synapses with other neurones). During the outgrowth of axonal processes the earliest nerve fibres are known to traverse appreciable distances over an apparently virgin landscape, often occupied by loose mesenchyme. A central problem for neurobiologists, therefore, has been understanding the mechanisms of axon guidance (Gordon-Weeks 2000). Axon guidance is thought to involve short-range, local guidance cues and long-range diffusible cues, any of which can be either attractive and permissive for growth, or repellent and hence inhibitory. Short-range cues require factors which are displayed on cell surfaces or in the extracellular matrix, e.g. axon extension requires a permissive, physical substrate, the molecules of which are actively recognized by the growth cone. They also require negative cues which inhibit the progress of the growth cone. Long-range cues come from gradients of specific factors diffusing from distant targets, which cause neurones to turn their axons towards the source of the attractive signal. The evidence for this has come from in vitro co-culture studies. The floor plate of the developing spinal cord exerts a chemotropic effect on commissural axons that later cross it, whereas there is chemorepulsion of developing motor axons from the floor plate. These forces are thought to act in vivo in concert in a dynamic process to ensure the correct passage of axons to their final destinations and to mediate their correct bundling together en route.
INDUCTION AND PATTERNING OF THE BRAIN AND SPINAL CORD
The regional pattern of the nervous system is induced before and during neural tube closure. Early concepts about regional patterning envisaged that regionalization within mesenchymal populations which transmit inductive signals to the ectoderm impose a similar mosaic of positional values on the overlying neural plate. For example, transplantation of caudal mesenchyme beneath the neural plate in Amphibia induced spinal cord, whereas rostral mesenchyme induced brain, as assessed by the morphology of the neuroepithelial vesicles. However, later work indicated a more complex scenario in which organizer grafts from early embryos induced mainly head structures, while later grafts induced mainly trunk structures. Subsequent molecular data have tended to support a model in which neural-inducing factors released by the organizer such as noggin, chordin and follistatin, neuralize the ectoderm and promote a mainly rostral neural identity. Later secreted signals then act to caudalize this rostral neural tissue, setting up an entire array of axial values along the neural tube. Candidates for these later, caudalizing, signals have been shown to be retinoic acid, fibroblast growth factors and the WNT secreted proteins, which are present in the paraxial mesenchyme and later in its derivatives, the somites. This combination of signals does not seem to be sufficient to produce the most rostral, forebrain structures. Other secreted proteins resident in the rostralmost part of the earliest ingressing axial populations of endoderm and mesenchyme are also capable of inducing markers of forebrain identity from ectodermal cells (Withington, Beddington & Cooke 2001).
As the neural tube grows and is modified in shape, a number of mechanisms refine the crude rostrocaudal pattern which has been imposed during neurulation. Molecules which diffuse from tissues adjacent to the neural tube such as the somites have patterning influences. The neural tube possesses a number of intrinsic signalling centres, such as the midbrain–hindbrain boundary, which produce diffusible molecules capable of influencing tissue development at a distance. In this way extrinsic and intrinsic factors serve to subdivide the neural tube into a number of fairly large domains, on which local influences can then act. Domains are distinguished by their expression of particular transcription factors, which in many cases have been causally related to the development of particular regions. Examples of such genes are the Hox family which are expressed in the spinal cord and hindbrain, and the Dlx, Emx and Otx families of genes which are expressed in various regions of the forebrain. All of these are developmental control genes which lie high up in the hierarchy, and are capable of initiating cascades of expression of other genes to create a more fine-grained pattern of cellular differentiation. In contrast to the aforementioned secreted molecules, these genes encode proteins which are retained in the cell nucleus, and so can act on DNA to induce or repress further gene expression.
Segmentation in the neural tube
The early neural tube is visibly divided into segments, termed neuromeres, by shallow transverse folds which extend perpendicular to its long axis. Primary neuromeres can be identified at stage 9, and 16 secondary neuromeres are present at stage 14. They are especially noted in the rhombencephalon, where they are termed rhombomeres; they have now been shown to constitute crucial units of pattern formation. Domains of expression of developmental control genes abut rhombomere boundaries; single cell labelling experiments have revealed that cells within rhombomeres form segregated non-mixing populations (Fig. 24.16). The neural crest also shows intrinsic segmentation in the rhombencephalon, and is segregated into streams at its point of origin in the dorsal neural tube. This may represent a mechanism whereby morphogenetic specification of the premigratory neural crest cells is conveyed to the pharyngeal arches (see Fig. 12.4). Although these segmental units lose their morphological prominence with subsequent development, they represent the fundamental ground plan of this part of the neuraxis, creating a series of semi-autonomous units within which local variations in patterning can then develop. The consequences of early segmentation for events later in development, such as the formation of definitive neuronal nuclei within the brain stem, and of peripheral axonal projections remain to be explored.
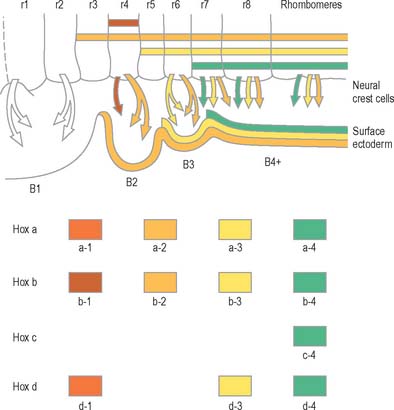
(Modified by permission from the Annual Review of Cell and Developmental Biology, Volume 8, 1992 by Annual Reviews www.annualreviews.org.)
Genes such as the Hox and Pax gene families, which encode transcription factor proteins, show intriguing expression patterns within the nervous system. Genes of the Hox-b cluster, for example, are expressed throughout the caudal neural tube, and up to discrete limits in the hindbrain that coincide with rhombomere boundaries. The ordering of these genes within a cluster on the chromosome (5′-3′) is the same as the caudal to rostral limits of expression of consecutive genes. This characteristic pattern is surprisingly similar in fish, frogs, birds and mammals. Hox genes play a role in patterning not only of the neural tube but also of much of the head region, consistent with their expression in neural crest cells, and within the pharyngeal arches. Disruption of Hox a-3 gene in mice mimics DiGeorge’s syndrome, a congenital human disorder characterized by the absence (or near absence) of the thymus, parathyroid and thyroid glands, by the hypotrophy of the walls of the arteries derived from the aortic arches, and by subsequent conotruncal cardiac malformations. Some Pax genes are expressed in different dorsoventral domains within the neural tube. Pax-3 is expressed in the alar lamina, including the neural crest, while Pax-6 is expressed in the intermediate plate. The Pax-3 gene has the same chromosomal localization as the mouse mutation Splotch and the affected locus in the human Waardenburg’s syndrome, both of which are characterized by neural crest disturbances with pigmentation disorders and occasional neural tube defects. Both Hox and Pax genes have restricted expression patterns with respect to the rostrocaudal and the dorsoventral axes of the neural tube, consistent with roles in positional specification. (For reviews of the expression patterns of these genes see Krumlauf et al 1993.)
While craniocaudal positional values are probably conferred on the neuroepithelium at the neural plate or early neural tube stage, dorsoventral positional values may become fixed later. The development of the dorsoventral axis is heavily influenced by the presence of the underlying notochord. The notochord induces the ventral midline of the neural tube, the floor plate. This specialized region consists of a strip of non-neural cells with distinctive adhesive and functional properties. Notochord and floor plate together participate in inducing the differentiation of the motor columns. Motor neurone differentiation occurs early, giving some grounds for the idea of a ventral to dorsal wave of differentiation. The notochord/floor plate complex may also be responsible for allotting the values of more dorsal cell types within the tube (Fig. 24.7). For example, the dorsal domain of expression of Pax-3 extends more ventrally in embryos experimentally deprived of notochord and floor plate, while grafting an extra notochord adjacent to the dorsal neural tube leads to a repression of Pax-3 expression.
PERIPHERAL NERVOUS SYSTEM
SOMATIC NERVES
Spinal nerves
Each spinal nerve is connected to the spinal cord by a ventral root and a dorsal root (Fig. 24.17). The fibres of the ventral roots grow out from cell bodies in the anterior and lateral parts of the intermediate zone. These pass through the overlying marginal zone and external limiting membrane. Some enter the myotomes of the somites, and some penetrate the somites, reaching the adjacent somatopleure, and in both sites they ultimately form the α-, β- and γ-efferents. At appropriate levels these are accompanied by the outgrowing axons of preganglionic sympathetic neuroblasts (segments T1–L2), or preganglionic parasympathetic neuroblasts (S2–S4).
The fibres of the dorsal roots extend from cell somata in dorsal root ganglia (DRG) into the spinal cord and also extend into the periphery. Neural crest cells are produced continuously along the length of the spinal cord, but gangliogenic cells migrate only into the rostral part of each somitic sclerotome where they condense and proliferate to form a bilateral series of oval-shaped primordial spinal ganglia (dorsal root ganglia) (Fig. 24.11). Negative factors in the caudal sclerotome deter neural crest from entering. The rostral sclerotome has a mitogenic effect on the crest cells that settle within it. From the ventral region of each ganglion a small part separates to form sympathochromaffin cells, while the remainder becomes a definitive spinal ganglion (dorsal root ganglion). The spinal ganglia are arranged symmetrically at the sides of the neural tube and, except in the caudal region, are equal in number to the somites. The cells of the ganglia, like the cells of the intermediate zone of the early neural tube, are glial and neuronal precursors. The glial precursors develop into satellite cells (which become closely applied to the ganglionic nerve cell somata), Schwann cells, and possibly other cells. The neuroblasts, at first round or oval, soon become fusiform, and their extremities gradually elongate into central and peripheral processes. The central processes grow into the neural tube as the fibres of dorsal nerve roots, while the peripheral processes grow ventrolaterally to mingle with the fibres of the ventral root, thus forming a mixed spinal nerve. As development proceeds the original bipolar form of the cells in the spinal ganglia changes and the two processes become approximated until they ultimately arise from a single stem to form a unipolar cell. The bipolar form is retained in the ganglion of the vestibulocochlear nerve.
Cranial nerves
Cranial nerves may contain motor, sensory or both types of fibres. With the exception of the olfactory and optic nerves, the cranial nerves develop in a manner similar in some respects to components of the spinal nerves. The somata of motor neuroblasts originate within the neuroepithelium, while those of sensory neuroblasts are derived from the neural crest with the addition in the head of contributions from ectodermal placodes (Fig. 24.18; see Fig. 12.4).
In the head the motor outflow is traditionally segregated into two pathways (Figs 24.3B and 24.18). General somatic efferent neurones exit ventrally in a similar manner to those of the spinal cord. Thus the oculomotor, trochlear, abducens and hypoglossal nerves parallel the organization of the somatic motor neurones in the spinal cord. The second motor component, special branchial efferent, consists of the motor parts of the trigeminal, facial, glossopharyngeal and vagus nerves which supply the pharyngeal (branchial) arches, and the accessory nerve. These nerves all have nerve exit points more dorsally placed than the somatic motor system.
These motor neurone types have been designated according to the types of muscles or structures they innervate. General somatic efferent nerves supply striated muscle derived from the cranial (occipital) somites and prechordal mesenchyme. Myogenic cells from the ventrolateral edge of the epithelial plate of occipital somites give rise to the intrinsic muscles of the tongue, while the prechordal mesenchyme gives rise to the extrinsic ocular muscles. Special branchial efferent nerves supply the striated muscles developing within the pharyngeal (branchial) arches (see Fig. 12.4) which are derived from parachordal mesenchyme between the occipital somites and the prechordal mesenchyme. All the voluntary muscles of the head originate from axial (prechordal) or paraxial mesenchyme which renders the distinction between somatic efferent supply and branchial efferent supply somewhat artificial. However, the obviously special nature of the arch musculature, its patterning by the neural crest cells, its particularly rich innervation for both voluntary and reflex activity, and the different origins from the basal plate of the branchial efferent nerves compared to the somatic efferent nerves, make the retention of a distinction between the two of some value.
The cranial sensory ganglia are derived in part from the neural crest, and in part from cells of the ectodermal placodes (Figs 24.12 and 24.18). Generally, neurones distal to the brain are derived from placodes while proximal ones are derived from the neural crest (Fig. 24.18). Supporting cells of all sensory ganglia arise from the neural crest. The most rostral sensory ganglion, the trigeminal, contains both neural crest and placode-derived neurones that mediate general somatic afferent functions. In the case of more caudal cranial nerves (the facial, glossopharyngeal and vagus), the same applies, but the two cell populations form separate ganglia in the case of each nerve. The proximal series of ganglia is neural crest derived (forming the proximal ganglion of the facial nerve, the superior ganglion of the glossopharyngeal nerve and the jugular ganglion of the vagus) while the distal series is derived from placodal cells (forming the geniculate ganglion of the facial nerve, the petrosal ganglion of the glossopharyngeal nerve and the nodose ganglion of the vagus). These ganglia contain neurones that mediate special, general visceral and somatic afferent functions. The vestibular ganglion contains both crest and placodal cells and the acoustic ganglion contains only placodal neurones: the axons from these cells are special somatic afferents and they all travel in the vestibulocochlear nerve.
AUTONOMIC NERVOUS SYSTEM
In the trunk at neurulation, neural crest cells migrate from the neural epithelium to lie transitorily on the fused neural tube. Thereafter crest cells migrate laterally and then ventrally to their respective destinations (Fig. 24.11). Within the head the neural crest cells migrate prior to neural fusion, producing a vast mesenchymal population as well as autonomic neurones.
Neurones of the enteric nervous system are described as arising from the vagal crest, i.e. neural crest derived from somite levels 1–7, and the sacral crest, caudal to the 28th somite. At all of these levels the crest cells also differentiate into glial-like support cells alongside the neurones (Fig. 24.19).
Parasympathetic ganglia
Neural crest cells from the caudal third of the mesencephalon and the rostral metencephalon migrate along or close to the ophthalmic branch of the trigeminal nerve and give rise to the ciliary ganglion. Cells migrating from the nucleus of the oculomotor nerve may also contribute to the ganglion; a few scattered cells are always demonstrable in postnatal life along the course of this nerve. Preotic myelencephalic neural crest cells give rise to the pterygopalatine ganglion, which may also receive contributions from the ganglia of the trigeminal and facial nerves. The otic and submandibular ganglia are also derived from myelencephalic neural crest and may receive contributions from the glossopharyngeal and facial cranial nerves respectively (see Fig. 12.4).
Sympathetic ganglia
Neural crest cells migrate ventrally within the body segments, penetrate the underlying somites and continue to the region of the future paravertebral and prevertebral plexuses, where they form the sympathetic chain of ganglia and the major ganglia around the ventral visceral branches of the abdominal aorta (Figs 24.11 and 24.19). Neural crest cells are induced to differentiate into sympathetic neurones by the dorsal aorta through the actions of the signaling molecules, Bmp-4 and Bmp-7.
Enteric nervous system
The enteric nervous system is derived from the neural crest. The axial levels of crest origin are shown in Fig. 24.19. Premigratory neural crest cells are not prepatterned for specific axial levels, rather they attain their axial value as they leave the neuraxis. Once within the gut wall there is a regionally specific pattern of enteric ganglia formation which may be controlled by the local splanchnopleuric mesenchyme. Cranial neural crest from somite levels 1–7 contributes to the enteric nervous system, forming both neuroblasts and glial support cells.
CENTRAL NERVOUS SYSTEM
SPINAL CORD
The neuroblasts of the lateral walls of the tube are large and at first round or oval (apolar). Soon they develop processes at opposite poles and become bipolar neurons. However, one process is withdrawn and the neuroblast becomes unipolar, although this is not invariably so in the case of the spinal cord. Further differentiation leads to the development of dendritic processes and the cells become typical multipolar neurones. In the developing cord they occur in small clusters representing clones of neurones. The development of a longitudinal sulcus limitans on each side of the central canal of the cord divides the ventricular and intermediate zones in each lateral wall into a basal (ventrolateral) plate or lamina and an alar (dorsolateral) plate or lamina (Fig. 24.17). This separation indicates a fundamental functional difference. Neural precursors in the basal plate include the motor cells of the anterior (ventral) and lateral grey columns, while those of the alar plate exclusively form ‘interneurones’ (which possess both short and long axons), some of which receive the terminals of primary sensory neurones. Caudally the central canal of the cord ends as a fusiform dilatation, the terminal ventricle.
Anterior (ventral) grey column
The cells of the ventricular zone are closely packed at this stage and arranged in radial columns (Fig. 24.6). Their disposition may be determined in part by contact guidance along the earliest radial array of glial fibres which cross the full thickness of the early neuroepithelium. The cells of the intermediate zone are more loosely packed. They increase in number initially in the region of the basal plate. This enlargement outlines the anterior (ventral) column of the grey matter and causes a ventral projection on each side of the median plane: the floor plate remains at the bottom of the shallow groove so produced. As growth proceeds these enlargements, which are further increased by the development of the anterior funiculi (tracts of axons passing to and from the brain), encroach on the groove until it becomes converted into the slit-like anterior median fissure of the adult spinal cord (Fig. 24.17). The axons of some of the neuroblasts in the anterior grey column cross the marginal zone and emerge as bundles of ventral spinal nerve rootlets on the anterolateral aspect of the spinal cord. These constitute, eventually, both the α-efferents which establish motor end plates on extrafusal striated muscle fibres and the γ-efferents which innervate the contractile polar regions of the intrafusal muscle fibres of the muscle spindles.
Posterior (dorsal) grey column
The posterior (dorsal) column develops later; consequently the ventricular zone is for a time much thicker in the dorsolateral lamina (alar plate) than it is in the ventrolateral lamina (basal plate) (Fig. 24.6).
While the columns of grey matter are being defined, the dorsal region of the central canal becomes narrow and slit-like, and its walls come into apposition and fuse with each other (Fig. 24.17). In this way the central canal becomes relatively reduced in size and somewhat triangular in outline.
About the end of the fourth week advancing axonal sprouts invade the marginal zone. The first to develop are those destined to become short intersegmental fibres from the neuroblasts in the intermediate zone, and fibres of dorsal roots of spinal nerves which pass into the spinal cord from neuroblasts of the early spinal ganglia. The earlier dorsal root fibres that invade the dorsal marginal zone arise from small dorsal root ganglionic neuroblasts. By the sixth week they form a well-defined oval bundle near the peripheral part of the dorsolateral lamina (Figs 24.6 and 24.7). This bundle increases in size and, spreading towards the median plane, forms the primitive posterior funiculus of fine calibre. Later, fibres derived from new populations of large dorsal root ganglionic neuroblasts join the dorsal root: they are destined to become fibres of much larger calibre. As the posterior funiculi increase in thickness, their medial surfaces come into contact separated only by the posterior medial septum, which is ependymal in origin and neuroglial in nature. It is thought that the displaced primitive posterior funiculus may form the basis of the dorsolateral tract or fasciculus (of Lissauer).
BRAIN
A summary of the derivatives of the cerebral regions from caudal to rostral is given in Table 24.1.
Rhombencephalon
By the time the midbrain flexure appears, the length of the rhombencephalon is greater than that of the combined extent of the mesencephalon and prosencephalon. Rostrally it exhibits a constriction, the isthmus rhombencephali (Fig. 24.3B), best viewed from the dorsal aspect. Ventrally the hindbrain is separated from the dorsal wall of the primitive pharynx only by the notochord, the two dorsal aortae and a small amount of mesenchyme; on each side it is closely related to the dorsal ends of the pharyngeal arches.
The pontine flexure appears to ‘stretch’ the thin, epithelial roof plate which becomes widened. The greatest increase in width corresponds to the region of maximum convexity, so that the outline of the roof plate becomes rhomboidal. By the same change the lateral walls become separated, particularly dorsally, and the cavity of the hindbrain, subsequently the fourth ventricle, becomes flattened and somewhat triangular in cross-section. The pontine flexure becomes increasingly acute until, at the end of the second month, the laminae of its cranial (metencephalic) and caudal (myelencephalic) slopes are opposed to each other (see Fig. 24.21) and, at the same time, the lateral angles of the cavity extend to form the lateral recesses of the fourth ventricle.
At about four and a half weeks of development, when the pontine flexure is first discernible, the association between the rhombomeres and the underlying motor nuclei of certain cranial nerves can be seen. The general pattern of distribution of motor nuclei is as follows: rhombomere 1 contains the trochlear nucleus, rhombomeres 2 and 3 contain the trigeminal nucleus, rhombomeres 4 and 5 contain the facial nucleus, rhombomere 5 contains the abducens nucleus, rhombomeres 6 and 7 contain the glossopharyngeal nucleus, and rhombomeres 7 and 8 contain the vagal, accessory and hypoglossal nuclei. Rhombomeric segmentation represents the ground plan of development in this region of the brain stem and is pivotal for the development of regional identity (see Fig. 12.4). However, with further morphogenesis the obvious constrictions of the rhombomere boundaries disappear, and the medulla once again assumes a smooth contour. The differentiation of the lateral walls of the hindbrain into basal (ventrolateral) and alar (dorsolateral) plates has a similar significance to the corresponding differentiation in the lateral wall of the spinal cord, and ventricular, intermediate and marginal zones are formed in the same way.
Cells of the basal plate (ventrolateral lamina)
Cells of the basal plate form three elongated, discontinuous, columns that are positioned ventrally and dorsally with an intermediate column between (Fig. 24.20).
Cell columns of the alar plate (dorsolateral lamina)
Cell columns of the alar plate are discontinuous and give rise to general visceral (general splanchnic) afferent, special visceral (special splanchnic) afferent, general somatic afferent, and special somatic afferent nuclei (their relative positions, in simplified transverse section, are shown in Fig. 24.20). The general visceral afferent column is represented by a part of the dorsal nucleus of the vagus, the special visceral afferent column by the nucleus of the tractus solitarius, the general somatic afferent column by the afferent nuclei of the trigeminal nerve and the special somatic afferent column by the nuclei of the vestibulocochlear nerve. (The relatively simple functional independence of these afferent columns implied by the foregoing classification is, in the main, an aid to elementary learning. The emergent neurobiological mechanisms are in fact much more complex and less well understood.) Although they tend to retain their primitive positions, some of these nuclei are later displaced by differential growth patterns and by the appearance and growth of neighbouring fibre tracts, and possibly by active migration.
Myelencephalon
The caudal slope of the embryonic hindbrain constitutes the myelencephalon, which develops into the medulla oblongata (Fig. 24.2). The nuclei of the ninth, tenth, 11th and 12th cranial nerves develop in the positions already indicated and afferent fibres from the ganglia of the ninth and tenth nerves form an oval marginal bundle in the region overlying the alar (dorsolateral) lamina. Throughout the rhombencephalon, the dorsal edge of this lamina is attached to the thin expanded roof plate and is termed the rhombic lip. (The inferior rhombic lip is confined to the myelencephalon; the superior rhombic lip to the metencephalon.) As the walls of the rhombencephalon spread outwards, the rhombic lip protrudes as a lateral edge which becomes folded over the adjoining area. The rhombic lip may later become adherent to this area, and its cells migrate actively into the marginal zone of the basal plate. In this way the oval bundle which forms the tractus solitarius becomes buried. Alar plate cells which migrate from the rhombic lip are believed to give rise to the olivary and arcuate nuclei and the scattered grey matter of the nuclei pontis. While this migration is in progress, the floor plate is invaded by fibres which cross the median plane (accompanied by neurones that cluster in and near this plane), and it becomes thickened to form the median raphe. Some of the migrating cells from the rhombic lip in this region do not reach the basal plate and form an oblique ridge, the corpus pontobulbare (nucleus of the circumolivary bundle), across the dorsolateral aspect of the inferior cerebellar peduncle.
Metencephalon
The rostral slope of the embryonic hindbrain is the metencephalon, from which both the cerebellum and pons develop. Before formation of the pontine flexure, the dorsolateral laminae of the metencephalon are parallel with one another. After its formation the roof plate of the hindbrain becomes rhomboidal and the dorsal laminae of the metencephalon lie obliquely. They are close at the cranial end of the fourth ventricle, but widely separated at the level of its lateral angles (see Fig. 24.21). Accentuation of the flexure approximates the cranial angle of the ventricle to the caudal, and the alar plates of the metencephalon now lie almost horizontally.
Fourth ventricle and choroid plexus
Caudal to the developing cerebellum the roof of the fourth ventricle remains epithelial, and covers an approximately triangular zone from the lateral angles of the rhomboid fossa to the median obex (see Fig. 24.21). Nervous tissue fails to develop over this region and vascular pia mater is closely applied to the subjacent ependyma. At each lateral angle and in the midline caudally the membranes break through forming the lateral (Luschka) and median (Magendie) apertures of the roof of the fourth ventricle. These become the principal routes by which cerebrospinal fluid, produced in the ventricles, escapes into the subarachnoid space. The vascular pia mater (tela choroidea), in an inverted V formation cranial to the apertures, invaginates the ependyma to form vascular fringes which become the vertical and horizontal parts of the choroid plexuses of the fourth ventricle.
Cerebellum
The cerebellum develops from the rhombic lip, the dorsal part of the alar plate of the metencephalon, which constitutes the rostral margin of the diamond-shaped fourth ventricle. Two rounded swellings develop which at first project partly into the ventricle (Fig. 24.21), forming the rudimentary cerebellar hemispheres. The most rostral part of the roof of the metencephalon originally separates the two swellings, but it becomes invaded by cells derived from the alar plate, which form the rudiments of the vermis. At a later stage, extroversion of the cerebellum occurs, its intraventricular projection is reduced and the dorsal extraventricular prominence increases. The cerebellum now consists of a bilobar (dumb-bell shaped) swelling stretched across the rostral part of the fourth ventricle (Fig. 24.21). It is continuous rostrally with the superior medullary velum, formed from the isthmus rhombencephali, and caudally with the epithelial roof of the myelencephalon. With growth, a number of transverse grooves appear on the dorsal aspects of the cerebellar rudiment: these are the precursors of the numerous fissures which characterize the surface of the mature cerebellum (Fig. 24.22).
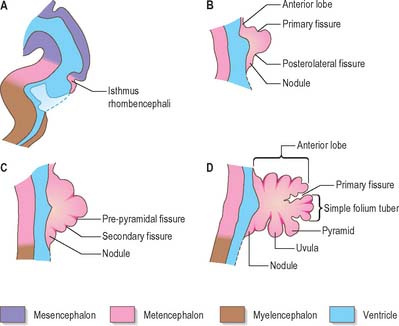
Fig. 24.22 Median sagittal sections through the developing cerebellum, at four chronologically later stages.
The first fissure to appear on the cerebellar surface (Fig. 24.22) is the lateral part of the posterolateral fissure which forms the border of a caudal region corresponding to the flocculi of the adult. The right and left parts of this fissure subsequently meet in the midline, where they form the boundary between the most caudal vermian lobule, the nodule, and the rest of the vermis. The flocculonodular lobe can now be recognized as the most caudal cerebellar subdivision at this stage and it serves as the attachment of the epithelial roof of the fourth ventricle. Because of the expansion of the other divisions of the cerebellum, the flocculonodular lobe comes to occupy an anteroinferior position in adults. At the end of the third month a transverse sulcus appears on the rostral slope of the cerebellar rudiment and deepens to form the fissura prima. This cuts into the vermis and both hemispheres, and forms the border between the anterior and posterior lobes. Contemporaneously, two short transverse grooves appear in the caudal vermis. The first is the fissura secunda (postpyramidal fissure), which forms the rostral border of the uvula; the second, the prepyramidal fissure, demarcates the pyramid (Fig. 24.22). The cerebellum now grows dorsally, rostrally, caudally and laterally, and the hemispheres expand much more than the inferior vermis, which therefore becomes buried at the bottom of a deep hollow, the vallecula. Numerous other transverse grooves develop, the most extensive being the horizontal fissure.
Cellular development of the cerebellum
The developed cerebellar cortex contains three layers, namely the molecular layer, the Purkinje layer, and the granular layer. The early bilateral expansion of the ventricular surface reflects the production, by the metencephalic alar plate ventricular epithelium, of neuroblasts which will give rise to the radial glia, cerebellar nuclei, and efferent neurones of the cerebellar cortex (the Purkinje cells) (Fig. 24.23). The radial glia play a role in guiding the Purkinje cells to the meningeal surface of the cerebellar anlage. During this early stage of cerebellar development, which is dominated by the production and migration of efferent cerebellar neurones, the surface of the cerebellar anlage remains smooth. The extroversion of the cerebellum begins later when cells of the external germinative layer, also termed the superficial matrix, begin proliferation and migration. These cells produce the granule cells, which migrate inward along the radial glia, through the layers of Purkinje cells, settling deep to them in the granular layer. This stage coincides with the emergences of the transverse folial pattern. Proliferation and migration of granule cells leads to a great rostrocaudal expansion of the meningeal surface of the cerebellum, forming the transverse fissures and transforming the multicellular layer of Purkinje cells into a monolayer. Purkinje cells and nuclear cells are formed prior to the granule cells, and granule cells serve as the recipient of the main afferent (mossy fibre) system of the cerebellum. Thus the development of the efferent neurones of the cerebellar cortex and nuclei precedes the development of its afferent organization.
When the external germinative layer is initially formed, the multicellular Purkinje cell layer beneath is not uniform, but subdivided into clusters which form rostrocaudally extending columns (Fig. 24.24). The medial Purkinje cell clusters develop into the future vermis. These Purkinje cells will grow axons which connect to neurones in the vestibular nuclei and the fastigial nucleus. The lateral clusters belong to the future hemispheres and will grow axons terminating in the interposed and dentate nuclei. The sharp border in the efferent projections from the vermis and hemispheres is thus established at an early age. These clusters will give rise to Purkinje cell zones in the adult cerebellum which project to a single vestibular or cerebellar nucleus.
In the developing human brain only the external germinative layer can be seen at 17–18 weeks; the Purkinje cells become apparent between 20–23 weeks. After 30 weeks four layers can be recognized, the extermal germinative layer (external granular layer) is formed by 6–8 rows of densely packed small round cells; the Purkinje cell layer is formed by 5–6 layers of larger, round immature neurones external to the internal granular layer; the molecular layer contains cells resembling external granular layer cells in migration (Lavezzi et al 2006). The external granular layer involutes between 5 and 7 months after birth: it is only a discontinuous layer at 10 months, and is totally absent by 12 months, after which time the cerebellar cortex shows a three-layered structure. From 5 to 7 months the Purkinje cells are reduced in number, more widely spaced and display mature polygonal somata with evident axon and dendrites.
Mesencephalon
The mesencephalon or midbrain is subdivided early in development into two neuromeres, mesencephalon 1 and mesencephalon 2. It persists for a time as a thin-walled tube enclosing a cavity of some size, separated from that of the prosencephalon by a slight constriction and from the rhombencephalon by the isthmus rhombencephali (Figs 24.2 and 24.25). Later, its cavity becomes relatively reduced in diameter, and in the adult brain it forms the cerebral aqueduct. The basal (ventrolateral) plate of the midbrain increases in thickness to form the cerebral peduncles, which are at first of small size, but enlarge rapidly after the fourth month, when their numerous fibre tracts begin to appear in the marginal zone. The neuroblasts of the basal plate of mesencephalon 2 give rise to the nuclei of the oculomotor nerve and some grey masses of the tegmentum, while the nucleus of the trochlear nerve remains in the region of the isthmus rhombencephali. The cells which give rise to the trigeminal mesencephalic nucleus arise either side of the dorsal midline, from the isthmus rhobencephali rostrally across the roof of the mesencephalon. Recent studies have shown that the progenitors of these cells do not express neural crest cell markers.
Prosencephalon
At an early stage, a transverse section through the forebrain shows the same parts as are displayed in similar sections of the spinal cord and medulla oblongata, i.e. thick lateral walls connected by thin floor and roof plates. Moreover, each lateral wall is divided into a dorsal area and a ventral area separated internally by the hypothalamic sulcus (Fig. 24.25). This sulcus ends rostrally at the medial end of the optic stalk. In the fully developed brain it persists as a slight groove extending from the interventricular foramen to the cerebral aqueduct. It is analogous to, if not the homologue of, the sulcus limitans. The thin roof plate remains epithelial, but invaginated by vascular mesenchyme, the tela choroidea of the choroid plexuses of the third ventricle. Later, the lateral margins of the tela undergo a similar invagination into the medial walls of the cerebral hemispheres. The floor plate thickens as the nuclear masses of the hypothalamus and subthalamus develop.
At a very early period, before the closure of the rostral neuropore, the subdivision of the prosencephalon into the most rostral telencephalon and two subdivisions of the diencephalon, D1 and D2, is heralded (Fig. 24.2). At this early time two eye fields are separated by the future neurohypophysis in the floor of the future D1. After head folding, the eye fields expand as two lateral optic evaginations which become optic vesicles, one on each side of the early brain. For a time they communicate with the cavity of the prosencephalon by relatively wide openings. The distal parts of the optic vesicles expand, while the proximal parts become the tubular optic stalks. The optic vesicles (which are described with the development of the eye in Ch. 41) are thus derived from the lateral walls of the D1 subdivision of the prosencephalon before the telencephalon can be clearly identified. The optic chiasma is often regarded as the boundary between diencephalon and telencephalon.
As the most rostral portion of the prosencephalon enlarges it curves ventrally, and two further diverticula expand rapidly from it, one on each side. These diverticula, which are rostrolateral to the optic stalks, subsequently form the cerebral hemispheres. Their cavities are the rudiments of the lateral ventricles and they communicate with the median part of the forebrain cavity by relatively wide openings which ultimately become the interventricular foramina. The rostral limit of the median part of the forebrain consists of a thin sheet, the lamina terminalis (Fig. 24.25A–C), which stretches from the interventricular foramina to the recess at the base of the optic stalks. The rostral part of the forebrain, including the rudiments of the cerebral hemispheres, consists of the telencephalon and the caudal part of the diencephalon: both contribute to the formation of the third ventricle, although the latter predominates. The fate of the lamina terminalis is described below.
Diencephalon
The diencephalon, D2, is broadly divided by the hypothalamic sulcus into dorsal (pars dorsalis diencephali) and ventral (pars ventralis diencephali) parts: each contributes to diverse neural structures. The dorsal part develops into the (dorsal) thalamus and metathalamus along the immediate suprasulcal area of its lateral wall, while the highest dorsocaudal lateral wall and roof form the epithalamus. The thalamus (Fig. 24.25A–C) is first visible as a thickening that involves the rostral part of the dorsal area. Caudal to the thalamus, the lateral and medial geniculate bodies, or metathalamus, are recognizable at first as surface depressions on the internal aspect and as elevations on the external aspect of the lateral wall. As the thalami enlarge as smooth ovoid masses, the wide interval between them gradually narrows into a vertically compressed cavity which forms the greater part of the third ventricle. After a time these medial surfaces may come into contact and become adherent over a variable area, the connection (single or multiple) constituting the interthalamic adhesion or massa intermedia. The caudal growth of the thalamus excludes the geniculate bodies from the lateral wall of the third ventricle.
At first the lateral aspect of the developing thalamus is separated from the medial aspect of the cerebral hemisphere by a cleft, but with growth the cleft becomes obliterated (Fig. 24.26) as the thalamus fuses with the part of the hemisphere in which the corpus striatum is developing. Later, with the development of the projection fibres (corticofugal and corticopetal) of the neocortex, the thalamus becomes related to the internal capsule, which intervenes between it and the lateral part of the corpus striatum (lentiform nucleus). Ventral to the hypothalamic sulcus, the lateral wall of the diencephalon, in addition to median derivatives of its floor plate, forms a large part of the hypothalamus and subthalamus.
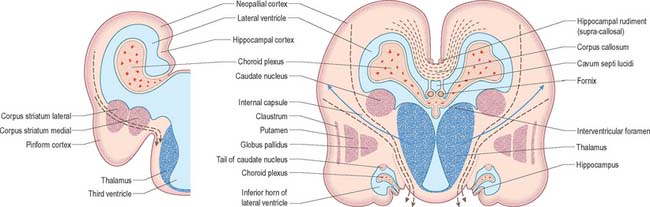
Fig. 24.26 The development of the basal nuclei and internal capsule, coronal views
(Redrawn by permission from Hamilton WJ, Boyd JD, Mossman HW 1972 Human Embryology: Prenatal Development of Form and Function. Baltimore: Williams and Wilkins.)
The ventral part of the diencephalon forms the subsulcal lateral walls of the third ventricle and takes part in the formation of the hypothalamus, including the mammillary bodies, the tuber cinereum and infundibulum of the hypophysis. The mammillary bodies arise as a single thickening which becomes divided by a median furrow during the third month. The tuber cinereum develops rostral to the mammillary bodies as a cellular proliferation that extends forwards as far as the infundibulum. In front of the tuber cinereum, a wide-mouthed diverticulum forms in the floor of the diencephalon, grows towards the stomodeal roof and comes into contact with the posterior aspect of a dorsally directed ingrowth from the stomodeum (Rathke’s pouch). These two diverticula together form the hypophysis cerebri (Fig. 24.14). An extension of the third ventricle persists in the base of the neural outgrowth as the infundibular recess. The remaining caudolateral walls and floor of the ventral diencephalon are an extension of the midbrain tegmentum, the subthalamus. This forms the rostral limits of the red nucleus, substantia nigra, numerous reticular nuclei and a wealth of interweaving, ascending, descending and oblique nerve fibre bundles, which have many origins and destinations.
Third ventricle and choroid plexus
The roof plate of the diencephalon rostral to the pineal gland, and continuing over the median telencephalon, remains thin and epithelial in character and is invaginated by the choroid plexuses of the third ventricle (Fig. 24.27). Before the development of the corpus callosum and the fornix it lies at the bottom of the longitudinal fissure, between and reaching the two cerebral hemispheres. It extends as far rostrally as the interventricular foramina and lamina terminalis. Here, and elsewhere, choroid plexuses develop by the close apposition of vascular pia mater and ependyma without intervening nervous tissue. With development, the vascular layer is infolded into the ventricular cavity and develops a series of small villous projections, each covered by a cuboidal epithelium derived from the ependyma. The cuboidal cells display numerous microvilli on their ventricular surfaces and complex folding of their basal plasma membranes. The early choroid plexuses secrete a protein-rich cerebrospinal fluid into the ventricular system which may provide a nutritive medium for the developing epithelial neural tissues. As the latter become increasingly vascularized the histochemical reactions of the cuboidal cells and the character of the fluid change to the adult type. Many regions of the lining of the third ventricle become highly specialized, and develop concentrations of tanycytes or other modified cells that are collectively termed the circumventricular organs, e.g. the subfornical organ, the organum vasculosum (intercolumnar tubercle) of the lamina terminalis, the subcommissural organ and the linings of the pineal, suprapineal, and infundibular recesses.
Telencephalon
The telencephalon consists of two lateral diverticula connected by a median region, the telencephalon impar. The rostral part of the third ventricle develops from the impar, and is closed below and in front by the lamina terminalis. The lateral diverticula are outpouchings of the lateral walls of the telencephalon, which may correspond to the alar lamina, although this is uncertain. Their cavities are the future lateral ventricles, and their walls are formed by the presumptive nervous tissue of the cerebral hemispheres. The roof plate of the median part of the telencephalon remains thin and is continuous behind with the roof plate of the diencephalon (Fig. 24.25). The rostral parts of the hypothalamus, which include the optic chiasma, optic recess and related nuclei, develop in the floor plate and lateral walls of the prosencephalon, ventral to the primitive interventricular foramina. The chiasma is formed by the meeting, and partial decussation, of the optic nerves in the ventral part of the lamina terminalis. The optic tracts subsequently grow backwards from the chiasma to end in the diencephalon and midbrain.
Cerebral hemispheres
The cerebral hemispheres arise as diverticula of the lateral walls of the telencephalon, with which they remain in continuity around the margins of initially relatively large interventricular foramina, except caudally, where they are continuous with the rostral part of the lateral wall of the diencephalon (Figs 24.2 and 24.25). As growth proceeds each hemisphere enlarges forwards, upwards and backwards and acquires an oval outline, medial and superolateral walls, and a floor. As a result the medial surfaces approach, but are separated by, a vascularized mesenchyme and pia mater which fills the median longitudinal fissure (Fig. 24.27). At this stage the floor of the fissure is the epithelial roof plate of the telencephalon, which is directly continuous caudally with the epithelial roof plate of the diencephalons.
At the early oval stage of hemispheric development, regions are named according to their future principal derivatives. The rostromedial and ventral floor becomes linked with the forming olfactory apparatus and is termed the primitive olfactory lobe. The floor (ventral wall, or base) of the remainder of each hemisphere forms the anlage of the primitive corpus striatum and amygdaloid complex, including its associated rim of lateral and medial walls (the striate part of the hemisphere). The rest of the hemisphere i.e. the medial, lateral, dorsal and caudal regions, is the suprastriate part of the hemisphere. Although it is the largest in terms of surface area, initially it possesses comparatively thin walls. The rostral end of each oval hemisphere becomes the definitive frontal pole. As the hemisphere expands, its original posterior pole moves relatively in a caudoventral and lateral direction, following a curve like a ram’s horn: it curves towards the orbit in association with the growth of the caudate nucleus and other structures to form the definitive temporal pole. A new posterior part persists as the definitive occipital pole of the mature brain (Fig. 24.28).
Olfactory bulb
A longitudinal groove appears in the anteromedial part of the floor of each developing lateral ventricle about the fifth week of embryonic development. It deepens and forms a hollow diverticulum which is continuous with the hemisphere by a short stalk. The diverticulum becomes connected on its ventral or inferior surface to the olfactory placode. Placodal cells give rise to afferent axons which terminate in the walls of the diverticulum. As the head increases in size, the diverticulum grows forwards, loses its cavity and becomes converted into the solid olfactory bulb. The forward growth of the bulb is accompanied by elongation of its stalk, which forms the olfactory tract. The part of the floor of the hemisphere to which the tract is attached constitutes the piriform area.
Lateral ventricles and choroid plexus
The early diverticulum or anlage of the cerebral hemisphere initially contains a simple spheroidal lateral ventricle which is continuous with the third ventricle via the interventricular foramen. The rim of the foramen is the site of the original evagination. The expanding ventricle develops the ram’s horn shape of the surrounding hemisphere, becoming first roughly ellipsoid and then a curved cylinder which is convex dorsally (Fig. 24.28). The ends of the cylinder expand towards, but do not reach, the frontal and (temporary) occipital poles; differentiating and thickening neural tissues separate the ventricular cavities and pial surfaces at all points, except along the line of the choroidal fissure. Pronounced changes in ventricular form accompany the emergence of a temporal pole. The original caudal end of the curved cylinder expands within its substance and the temporal extensions in each hemisphere pass ventrolaterally to encircle both sides of the upper brain stem. Another extension may develop from the root of the temporal extension in the substance of the definitive occipital pole and pass caudomedially; it is quite variable in size, often asymmetrical on the two sides, and one or both may be absent. Although the lateral ventricle is a continuous system of cavities, specific parts are now given regional names. The central part (body) extends from the interventricular foramen to the level of the posterior edge (splenium) of the corpus callosum. Three cornua (horns) diverge from the body: anterior towards the frontal pole, posterior towards the occipital pole, and inferior towards the temporal pole.
The pia mater which covers the epithelial roof of the third ventricle at this stage is itself covered with loosely arranged mesenchyme and developing blood vessels. These vessels subsequently invaginate the roof of the third ventricle on each side of the median plane to form its choroid plexuses. The lower part of the medial wall of the cerebral hemisphere, which immediately adjoins the epithelial roof of the interventricular foramen and the rostral extremity of the diencephalon, also remains epithelial. It consists of ependyma and pia mater; elsewhere the walls of the hemispheres are thickening to form the pallium. The thin part of the medial wall of the hemisphere is invaginated by vascular tissue which is continuous in front with the choroid plexus of the third ventricle and constitutes the choroid plexus of the lateral ventricle. This invagination occurs along a line which arches upwards and backwards, parallel with and initially limited to, the rostral and upper boundaries of the interventricular foramen. This curved indentation of the ventricular wall, where no nervous tissue develops between ependyma and pia mater, is termed the choroidal fissure (Figs 24.25C and 24.26). The subsequent assumption of the definitive form of the choroidal fissure depends on related growth patterns in neighbouring structures. Of particular importance are the relatively slow growth of the interventricular foramen, the secondary ‘fusion’ between the lateral diencephalon and medial hemisphere walls, the encompassing of the upper brain stem by the forward growth of the temporal lobe and its pole towards the apex of the orbit, and the massive expansion of two great cerebral commissures (the fornix and corpus callosum). The choroidal fissure is now clearly a caudal extension of the much reduced interventricular foramen, which arches above the thalamus and is here only a few millimetres from the median plane. Near the caudal end of the thalamus it diverges ventrolaterally, its curve reaching and continuing in the medial wall of the temporal lobe over much of its length (i.e. to the tip of the inferior horn of the lateral ventricle). The upper part of the arch will be overhung by the corpus callosum and, throughout its convexity, it is bordered by the fornix and its derivatives.
Basal nuclei
At first growth proceeds more actively in the floor and the adjoining part of the lateral wall of the developing hemisphere, and elevations formed by the rudimentary corpus striatum encroach on the cavity of the lateral ventricle (Figs 24.25 and 24.26). The head of the caudate nucleus appears as three successive parts, medial, lateral and intermediate, which produce elevations in the floor of the lateral ventricle. Caudally these merge to form the tail of the caudate nucleus and the amygdaloid complex, which both remain close to the temporal pole of the hemisphere. When the occipital pole grows backwards, and the general enlargement of the hemisphere carries the temporal pole downwards and forwards, the tail of the caudate is continued from the floor of the central part (body) of the ventricle into the roof of its temporal extension, the future inferior horn. The amygdaloid complex encapsulates its tip. Rostrally the head of the caudate nucleus extends forwards to the floor of the interventricular foramen, where it is separated from the developing rostral end of the thalamus by a groove; later, the head expands in the floor of the anterior horn of the lateral ventricle. The lentiform nucleus develops from two laminae of cells, medial and lateral, which are continuous with both the medial and lateral parts of the caudate nucleus. The internal capsule appears first in the medial lamina and extends laterally through the outer lamina to the cortex. It divides the laminae into two, the internal parts join the caudate nucleus and the external parts form the lentiform nucleus. In the latter, the remaining medial lamina cells give rise mainly to the globus pallidus and the lateral lamina cells to the putamen. The putamen subsequently expands concurrently with the intermediate part of the caudate nucleus.
Fusion of diencephalic and telencephalic walls
As the hemisphere enlarges, the caudal part of its medial surface overlaps and hides the lateral surface of the diencephalon (thalamic part), from which it is separated by a narrow cleft occupied by vascular connective tissue. At this stage (about the end of the second month) a transverse section made caudal to the interventricular foramen would pass from the third ventricular cavity successively through the developing thalamus, the narrow cleft just mentioned, the thin medial wall of the hemisphere, and the cavity of the lateral ventricle, with the corpus striatum in its floor and lateral wall (Fig. 24.26).
As the thalamus increases in extent it acquires a superior surface in addition to medial and lateral surfaces. The lateral part of its superior surface fuses with the thin medial wall of the hemisphere so that this part of the thalamus is finally covered with the ependyma of the lateral ventricle immediately ventral to the choroidal fissure. As a result the corpus striatum is approximated to the thalamus and is separated from it only by a deep groove which becomes obliterated by increased growth along the line of contact. The lateral aspect of the thalamus is now in continuity with the medial aspect of the corpus striatum so that a secondary union between the diencephalon and the telencephalon is affected over a wide area, providing a route for the subsequent passage of projection fibres to and from the cortex (Fig. 24.26).
Formation of the insula
At the end of the third month, while the corpus striatum is developing, there is a relative restriction of growth between the frontal and temporal lobes. The region lateral to the striatum becomes depressed to form a lateral cerebral fossa with a portion of cortex, the insula, at its base (Fig. 24.28). As the temporal lobe continues to protrude towards the orbit, and with more rapid growth of the temporal and frontal cortices, the surface of the hemisphere expands at a rate greater than the hemisphere as a whole and the cortical areas become folded, forming gyri and sulci. The insula is gradually overgrown by these adjacent cortical regions, and they overlap it forming the opercula, the free margins of which form the anterior part of the lateral fissure. This process is not completed until after birth. The lentiform nucleus remains deep to and coextensive with the insula.
Olfactory nerve, limbic lobe and hippocampus
The growth changes in the temporal lobe which help to submerge the insula produce important changes in the olfactory and neighbouring limbic areas. As it approaches the hemispheric floor, the olfactory tract diverges into lateral, medial and (variable) intermediate striae. The medial stria is clothed with a thin archaeocortical medial olfactory gyrus. This curves up into further archaeocortical areas rostral to the lamina terminalis (paraterminal gyrus, prehippocampal rudiment, parolfactory gyrus, septal nuclei) and these continue into the indusium griseum. The lateral stria, clothed by the lateral olfactory gyrus, and the intermediate stria (when present), terminate in the rostral parts of the piriform area, including the olfactory trigone and tubercle, anterior perforated substance, uncus and entorhinal area of the anterior part of the future parahippocampal gyrus. The lateral limit of the lateral stria is indicated by the rhinal sulcus. The forward growth of the temporal pole and the general expansion of the neocortex cause the lateral olfactory gyrus to bend laterally, the summit of the convexity lying at the anteroinferior corner of the developing insula (Fig. 24.29). During the fourth and fifth months, much of the piriform area becomes submerged by the adjoining neocortex and in the adult only a part of it remains visible on the inferior aspect of the cerebrum.
The limbic lobe is the first part of the cortex to differentiate and at first it forms a continuous, almost circular strip on the medial and inferior aspects of the hemisphere. Below and in front, where the stalk of the olfactory tract is attached, it constitutes a part of the piriform area. The portion outside the curve of the choroid fissure (Fig. 24.30) constitutes the hippocampal formation. In this region the neural progenitors of the developing cortex proliferate and migrate. The wall of the hemisphere thickens, producing an elevation that projects into the medial side of the ventricle. The elevation is the hippocampus; it appears first on the medial wall of the hemisphere in the area above and in front of the lamina terminalis (paraterminal area) and gradually extends backwards, curving into the region of the temporal pole where it adjoins the piriform area. The marginal zone in the neighbourhood of the hippocampus is invaded by neurones to form the dentate gyrus. Both extend from the paraterminal area backwards above the choroid fissure and follow its curve downwards and forwards towards the temporal pole, where they continue into the piriform area. A shallow groove, the hippocampal sulcus, crosses the medial surface of the hemisphere throughout the hippocampal formation. The efferent fibres from the cells of the hippocampus collect along its medial edge and run forwards immediately above the choroid fissure. Rostrally they turn ventrally and enter the lateral part of the lamina terminalis to gain the hypothalamus, where they end in and around the mammillary body and neighbouring nuclei. These efferent hippocampal fibres form the fimbria hippocampi and the fornix.
Projection fibres, internal capsule
The growth of the neocortex, and its enormous expansion during the latter part of the third month, is associated with the initial appearance of corticofugal and corticopetal projection fibres and the internal capsule. The fibres follow the route provided by the apposition of the lateral aspect of the thalamus with the medial aspect of the corpus striatum. They divide the latter, almost completely, into a lateral part, the lentiform nucleus, and a medial part, the caudate nucleus: these two nuclei remain confluent only in their anteroinferior regions (Figs 24.26 and 24.28). The corticospinal tracts begin to develop in the ninth week of fetal life and reach their caudal limits by the twenty-ninth week. The fibres destined for the cervical and upper thoracic regions, which innervate the upper limbs, are in advance of those which innervate the lower limbs, and these, in turn, are in advance of fibres that innervate the face: the timing of the appearance of reflexes associated with these three parts of the body is similarly staggered.
The majority of subcortical nuclear masses receive terminals from descending fibres of cortical origin. These are joined by thalamocortical, hypothalamocortical and other afferent ascending bundles. The internal capsular fibres pass lateral to the head and body of the caudate nucleus, the anterior cornu and central part of the lateral ventricle, the rostroventral extensions and body of the fornix, the dorsal thalamus and dorsal choroidal fissure, and medial to the lentiform nucleus (Fig. 24.26).
Formation of gyri and sulci
Apart from the shallow hippocampal sulcus and the lateral cerebral fossa, the surfaces of the hemisphere remain smooth and uninterrupted until early in the fourth month (Fig. 24.29). The parieto-occipital sulcus appears about that time on the medial aspect of the hemisphere. Its appearance seems associated with an increase in the number of splenial fibres in the corpus callosum. Over the same period the posterior part of the calcarine sulcus appears as a shallow groove extending forwards from a region near the occipital pole. It is a true infolding of the cortex in the long axis of the striate area and produces an elevation, the calcar avis, on the medial wall of the posterior horn of the ventricle.
During the fifth month the cingulate sulcus appears on the medial aspect of the hemisphere, and sulci appear on the inferior and superolateral aspects in the sixth month. The central, precentral and postcentral sulci appear, each in two parts, upper and lower, which usually coalesce shortly afterwards, although they may remain discontinuous. The superior and inferior frontal, the intraparietal, occipital, superior and inferior temporal, occipitotemporal, collateral and rhinal sulci all make their appearance during the same period. By the end of the eighth month all the important sulci can be recognized (Fig. 24.29).
Development of commissures
The development of the commissures causes a very profound alteration of the medial wall of the hemisphere. At the time of their appearance the two hemispheres are connected to each other by the median part of the telencephalon. The roof plate of this area remains epithelial, while its floor becomes invaded by the decussating fibres of the optic nerves and developing hypothalamic nuclei. These two routes are thus not available for the passage of commissural fibres passing from hemisphere to hemisphere across the median plane, and these fibres therefore pass through the rostral wall of the interventricular foramen, i.e. the lamina terminalis. The first commissures to develop are those associated with the palaeocortex and archicortex. Fibres of the olfactory tracts cross in the ventral or lower part of the lamina terminalis and, together with fibres from the piriform and prepiriform areas and the amygdaloid bodies, form the rostral part of the anterior commissure (Figs 24.30 and 24.31). In addition the two hippocampi become interconnected by transverse fibres which cross from fornix to fornix in the upper part of the lamina terminalis as the commissure of the fornix (hippocampal commissure). Various other decussating fibre bundles (known as the supraoptic commissures, although they are not true commissures) develop in the lamina terminalis immediately dorsal to the optic chiasma, between it and the anterior commissure.
The commissures of the neocortex develop later and follow the pathways already established by the commissures of the limbic system. Fibres from the tentorial surface of the hemisphere join the anterior commissure and constitute its larger posterior part. All the other commissural fibres of the neocortex associate themselves closely with the commissure of the fornix and lie on its dorsal surface. These fibres increase enormously in number and the bundle rapidly outgrows its neighbours to form the corpus callosum (Figs 24.30 and 24.31).
The corpus callosum originates as a thick mass connecting the two cerebral hemispheres around and above the anterior commissure. (This site has been called the precommissural area, but this use has been rejected here because of the increasing use of the adjective precommissural to denote the position of parts of the limbic lobe, i.e. prehippocampal rudiment, septal areas and nuclei and strands of the fornix, in relation to the anterior commissure of the mature brain.) The upper end of this neocortical commissural area extends backwards to form the trunk of the corpus callosum. The rostrum of the corpus callosum develops later and separates some of the rostral end of the limbic area from the remainder of the cerebral hemisphere. Further backward growth of the trunk of the corpus callosum then results in the entrapped part of the limbic area becoming stretched out to form the bilateral septum pellucidum. As the corpus callosum grows backwards it extends above the choroidal fissure, carrying the commissure of the fornix on its under surface. In this way a new floor is formed for the longitudinal fissure, and additional structures come to lie above the epithelial roof of the third ventricle. In its backward growth the corpus callosum invades the area hitherto occupied by the upper part of the archaeo-cortical hippocampal formation, and the corresponding parts of the dentate gyrus and hippocampus are reduced to vestiges, the indusium griseum and the longitudinal striae. However, the posteroinferior (temporal) archaeocortical regions of both dentate gyrus and hippocampus persist and enlarge.
Cellular development of the cerebrum
The wall of the earliest cerebral hemisphere consists of a pseudostratified epithelium, whose cells exhibit interkinetic nuclear migration as they proliferate to form clones of germinal cells. The columnar cells elongate and their non-nucleated peripheral processes now constitute a marginal zone (but see Fig. 24.32A legend), while their nucleated, paraluminal and mitosing regions constitute the ventricular zone. Some of their progeny leave the ventricular zone and migrate to occupy an intermediate zone. The proliferative phase continues for a considerable period of fetal life. Ultimately, groups of progenitor cells form, at first, generations of definitive neurones and, later, glial cells which migrate to, and mature in, their final positions. These phases of proliferation, migration, differentiation and maturation overlap each other in space and time, and are not precisely sequential.
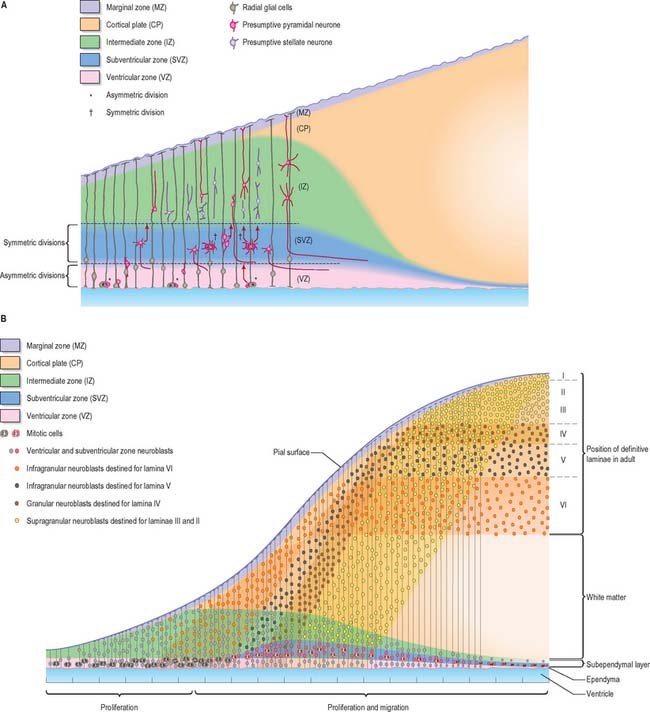
Fig. 24.32 A, Two distinct programmes of cell division are observed in the ventricular zone. Asymmetric division where radial glia give rise to neurones directly; symmetric division where neurones arise via intermediate progenitors after arrest in the subventricular zone. B, The dynamics of neuroblast proliferation and successive migrations to form the cerebral neocortex. Cells divide initially in the ventricular and subventricular zones and successive progeny migrate towards the pia. The first cells to migrate form deep cortical laminae; later cells pass through these layers to form successively superficial laminae. The following revisions to the 1970 Bolder Committee nomenclature for development of the human cerebral cortex given by Bystron, Blakemore and Rakic (2008) are included here, although not shown on the above diagram. Within the developing cerebral cortex: there is no distinct marginal zone beneath the pial layer before the cortical plate forms; the subventricular zone appears as a distinct proliferative layer before the cortical plate forms; a transient preplate layer forms before the cortical plate appears; the intermediate zone denotes a heterogenous compartment lying between the proliferative layers (ventricular and subventricular zones) and the postmigratory cells above; there is a transient subplate zone directly below the cortical plate.
(Adapted from Noctor et al 2004.)
The migration of neuronal precursors from the ventricular and intermediate zones occurs radially towards the pial surface Radial glial cells are now considered as neural progenitors (Kriegstein & Gotz 2003). Some early progeny from the ventricular zone form a primordial plexiform layer, or preplate. Their somata become arranged as a transient dense cortical plate from which subsequently generated cortical plate cells arise to form a subplate region. At later stages a considerable proportion of cortical progenitors divide in a subventricular zone (Fig. 24.32A). Proliferation in the ventricular zone, which produces excitatory pyramidal neurones of the infragranular layers of the cortex, wanes, whereas in the subventricular zone proliferation persists for a considerable period: the symmetrical terminal division of these cells gives rise to neurones which migrate to form the supragranular layers of the cortex (Kriegstein & Noctor 2004). From the pial surface inwards, the following zones may be defined: marginal, cortical plate, subplate, intermediate, subventricular and ventricular (Fig. 24.32B). (For a review of the nomenclature of the developing zones see Bystron et al 2008.) The marginal zone, the outermost layer of the developing cerebral cortex, will form layer 1, and the neurones of the cortical plate and subplate form the neurones of the remaining (2–6) cortical laminae, the complexity varying in different locations and with further additions of neurones from the deeper zones. The intermediate zone gradually transforms into the white matter of the hemisphere. Meanwhile other deep progenitor cells produce generations of glioblasts which also migrate into the more superficial layers. As proliferation wanes and finally ceases in the ventricular and subventricular zones, their remaining cells differentiate into general or specialized ependymal cells, tanycytes or subependymal glial cells.
In the pallial walls of the mammalian cerebral hemisphere, the phylogenetically oldest regions, which are the first to differentiate during ontogeny, are those that border the interventricular foramen and its extension the choroidal fissure, the lamina terminalis and the piriform lobe. The pallidum also contributes interneurones to the formation of the cerebral cortex. A large portion of GABAergic inhibitory (nonpyramidal) cells are not generated in the cortical ventricular zone, but migrate tangentially through the striatocortical junction to reach the cortex (Wonders & Anderson 2006; Metin et al 2006; Fig. 24.33). An increasingly complex level of organization, from three to six tangential laminae, is encountered in passing from the dentate gyrus and cornu ammonis through the subiculum to the general neocortex. (Many investigators find the simple progression from three to six major laminae a gross oversimplification, and numerous subdivisions have been proposed.)
Mechanisms of cortical development
Rakic (1971) initially demonstrated the migration of neurones along radial glial processes, and this has subsequently been seen to occur in three phases. First, the neurones become apposed to the radial glial cells and establish an axis of polarity away from the ventricular surface. Next, they are propelled along the glial surface until they ‘recognize’ their final destination, whereupon they cease locomotion and detach from the glial processes. They then continue to differentiate according to their final position, and later-born neurones migrate past them towards the pial surface (Fig. 24.5). Cortical neurones or cerebellar granule cells appear equally capable of migrating on hippocampal or cerebellar Bergmann glia, indicating conservation of migration mechanisms in different brain regions. These observations are still valid, although it is now recognized that radial glia represent the major neuronal progenitor population of the cortex.
In a plane perpendicular to its laminae, i.e. tangentially/circumferentially, the cortex is divided up into a number of areas, displaying a hierarchy of organization (see Ch. 23). These include primary areas, such as the motor cortex, unimodal association areas concerned with the integration of information from one of the primary areas, and multimodal association areas that integrate information from more than one modality. There are also the areas concerned with functions that are even less understood, such as the frontal lobes, concerned with goal-orientation, responsibility and long-term planning. The primary areas are further divided into somatotopic maps. At the finest level, the cortex is known to consist of a series of ‘columns’, 50–500 μm wide, within which cells on a vertical traverse display common features of modality and electrophysiological responses to stimuli, e.g. the ocular dominance columns of the visual cortex. Despite the precise stacking of neurones in these columns, only 80–85% of cells are thought to migrate radially along the glial cells: a subpopulation is thought to move tangentially in the intermediate zone (O’Rourke et al 1995). Moreover, some neurones may also migrate tangentially on the radial glial cells, as a result of glial cell branching in the cortical plate. The ventricular zone is not the only source of cortical neurones, because striatal and GABAergic neurones are known to migrate from the medial, lateral and caudal ganglionic eminences into the developing cortex. The proportion of locally generated and tangentially migrating GABAergic neurones it believed to be 65–35% in human and 5–95% in mouse.
Two models have been proposed to explain the development of the complexity of cortical organization. The ‘protocortex’ model assumes that the proliferative ventricular epithelium is a ‘tabula rasa’ generating homogeneous layers of neurones that are patterned solely by their future connectivity, especially by the ingrowth of processes from the thalamus. The ‘protomap’ hypothesis proposes that the intrinsic differences between the different areas are specified prior to cell generation and migration (Rakic 1988, 2003). The radial glial cells translate this map from the ventricular zone to the cortical plate where the pattern is refined by innervating axons. In this ‘radial unit’ model, the tangential co-ordinates of the different areas are determined by the position of their ventricular ancestors, whereas their radial position is determined by their time of birth and rate of migration.
But what would constitute such a ‘protomap’? The investigation of gene expression patterns shows that the early cortex is not homogeneous, and that it expresses some markers that are transient and some that persist into the adult. For example, the mouse gene Id2 marks the transition between the motor and somatosensory cortices in the embryo, whereas limbic associated membrane protein (LAMP) delineates the limbic cortex throughout life. LAMP expression is regulated by transforming growth factor-α, which is expressed by the lateral ganglionic eminence at the lateral edge of the cortex, whereas the medial edge or cortical hem expresses signalling molecules of the Wnt and BMP families. Any, if not all, of these may be the components of short range signalling centres along the edges of the cortex (e.g. FGF8) (Fig. 24.34). Coupled with the gradients of transcription factors such as Emx2 and Lhx2, there is therefore evidence to support the ‘protomap’ hypothesis (Donoghue & Rakic 1999). The challenge is to relate the gradients of gene expression patterns to formation of sharp cytoarchitectonic boundaries.
However, studies of cell migration are consistent with the idea that cortical areas might not be rigidly determined. Experimental manipulations of the developing cortex by deafferentation or manipulation of inputs give some indication of the state of commitment of cortical areas. In two independent sets of experiments, somatosensory or auditory cortex was induced to process visual information by misrouting retinal axons to somatosensory thalamus or auditory thalamus (von Melchner, Pallas & Sur 2000). When the lateral geniculate nucleus and the visual cortex were ablated and space was created in the medial geniculate by ablating the inferior colliculus, cells in the somatosensory or auditory cortex were visually driven due to the subcortical rewiring, and receptive field and response properties resembled those typical of the visual cortex. These results suggest that the modality of a sensory thalamic nucleus or cortical area can be specified by inputs during development.
Neonatal brain and reflexes
The sulci of the cerebral hemispheres appear from the fourth month of gestation (Fig. 24.29). At full term, the general arrangement of sulci and gyri is present, but the insula is not completely covered, the central sulcus is situated further rostrally, and the lateral sulcus is more oblique than in the adult. Most of the developmental stages of sulci and gyri have been identified in the brains of premature infants. Of the cranial nerves, the olfactory nerve and the optic nerve at the chiasma are much larger than in the adult, whereas the roots of the other nerves are relatively smaller.
Reflexes present at birth
A number of reflexes are present at birth and their demonstration is used to indicate normal development of the nervous system and responding muscles. Five tests of neurological development are most useful in determining gestational age. The pupillary reflex is consistently absent before 29 weeks’ gestation and present after 31 weeks; the glabellar tap, a blink in response to a tap on the glabella, is absent before 32 weeks and present after 34 weeks; the neck righting reflex appears between 34 and 37 weeks; the traction response, where flexion of the neck or arms occurs when the baby is pulled up by the wrists from the supine position appears after 33 weeks; head turning in response to light appears between 32 and 36 weeks. The spinal reflex arc is fully developed by the eighth week of gestation and lower limb flexor tone is detectable from about 29 weeks. The Babinski response, which involves extension of the great toe with spreading of the remaining toes in response to stimulation of the lateral aspect of the sole of the foot, is elicited frequently in neonates; it reflects poor cortical control of motor function by the immature brain. Generally reflexes develop as muscles gain tone. They appear in a sequential manner from caudal to cephalic, i.e. in the lower limb before the upper, and centripetally, i.e. distal reflexes appear before proximal ones (Allen & Capute 1990).
In full-term neonates, the placing of a spoon or food onto the anterior part of the tongue elicits an extrusion reflex: the lips are pursed and the tongue pushes vigorously against the object. By 4–6 months the reflex changes and food deposited on the anterior part of the tongue is moved to the back of the tongue, into the pharynx, and swallowed (see Ch. 33 for a description of swallowing in the adult). Rhythmic biting movements occur by 7–9 months postnatally, even in the absence of teeth.
MENINGES
The meninges may be divided in development into the pachymeninx (dura mater) and leptomeninges (arachnoid mater, subarachnoid space with arachnoid cells and fibres, and pia mater). All meningeal layers are derived from loose mesenchyme which surrounds the developing neural tube, termed meninx primitiva, or primary meninx. (For a detailed account of the development of the meninges in the human consult O’Rahilly & Müller 1986.)
The first indication of pia mater, containing a plexus of blood vessels which forms on the neural surface, is seen at stage 11 (24 days), around the caudal-most part of the medulla; this extends to the mesencephalic level by stage 12. Mesenchymal cells projecting from the rostral end of the notochord, and those in the region of the prechordal plate, extend rostrally into the mesencephalic flexure and form the earliest cells of the tentorium cerebelli; at the beginning of its development the medial part of the tentorium is predominantly leptomeningeal. By stage 17 (41 days) dura mater can be seen in the basal areas where the future chondrocranium is also developing. The precursors of the venous sinuses lie within the pachymeninx at stage 19 (48 days), and by stage 20, cell populations in the region of the future falx cerebri are proliferating, although the dorsal regions of the brain are not yet covered with putative meninges.
The growth of the cranial vault is initiated from ossification centres within the desmocranial mesenchyme. A wave of osteodifferentiation moves radially outward from these centres stopping when adjacent bones meet at regions where sutures are induced to form. Once sutures are formed, a second phase of development occurs in which growth of the cranial bones occurs at the sutural margins. This growth forms most of the skull (see Ch. 35). A number of hypotheses have been generated to explain the process of sutural morphogenesis. It has been suggested that the dura mater contains fibre tracts which extend from fixed positions in the cranial base to sites of dural reflection underlying each of the cranial sutures, and that the tensional forces so generated dictate the position of the sutures and locally inhibit precocious ossification. Other hypotheses support the concept of local factors in the calvaria which regulate suture morphogenesis. Following removal of the entire calvaria, the skull regenerates and sutures and bones develop in anatomically correct positions, suggesting that the dura can dictate suture position at least in regeneration of the neonatal calvaria. In transplants of sutures in which the fetal dura mater was left intact, a continuous fibrous suture remained between developing vault bones, whereas bony fusion occurred in transplants in which the fetal dura mater was removed (Opperman et al 1993).
VASCULAR SUPPLY
ARTERIES
Cranial arteries
The internal carotid artery is formed progressively from the third arch artery is the dorsal aorta cranial to this, and a further forward continuation which differentiates, at the time of regression of the first and second aortic arches, from the capillary plexus extending to the walls of the forebrain and midbrain. At its anterior extremity this primitive internal carotid artery divides into cranial and caudal divisions. The former terminates as the primitive olfactory artery, and supplies the developing regions implied (see Fig. 35.8). The latter sweeps caudally to reach the ventral aspect of the midbrain, its terminal branches are the primitive mesencephalic arteries. Simultaneously bilateral longitudinal channels differentiate along the ventral surface of the hindbrain from a plexus fed by intersegmental and transitory presegmental branches of the dorsal aorta and its forward continuation. The most important of the presegmental branches is closely related to the fifth nerve, the primitive trigeminal artery. Otic and hypoglossal presegmental arteries occur and may persist. The longitudinal channels later connect cranially with the caudal divisions of the internal carotid arteries (each of which gives rise to an anterior choroidal artery supplying branches to the diencephalon, including the telae choroideae and midbrain) and caudally with the vertebral arteries through the first cervical intersegmental arteries. Fusion of the longitudinal channels results in the formation of the basilar artery, while the caudal division of the internal carotid artery becomes the posterior communicating artery and the stem of the posterior cerebral artery. The remainder of the posterior cerebral artery develops comparatively late, probably from the stem of the posterior choroidal artery which is annexed by the caudally expanding cerebral hemisphere, its distal portion becoming a choroidal branch of the posterior cerebral artery. The posterior choroidal artery supplies the tela choroidea at the future temporal end of the choroidal fissure; its rami advance through the tela to become confluent with branches of the anterior choroidal artery. The cranial division of the internal carotid artery gives rise to anterior choroidal, middle cerebral and anterior cerebral arteries. The stem of the primitive olfactory artery remains as a small medial striate branch of the anterior cerebral artery. The cerebellar arteries, of which the superior is the first to differentiate, emerge from the capillary plexus on the wall of the rhombencephalon.
Vascularization of the brain
The leptomeningeal perforating branches pass into the brain parenchyma as cortical, medullary and striate branches (Fig. 24.35). The cortical vessels supply the cortex via short branches which may form precapillary anastomoses, whereas the medullary branches supply the white matter. The latter converge towards the ventricle but rarely reach it; they often follow a tortuous course as they pass around bundles of nerves. The striate branches which penetrate into the brain through the anterior perforated substance, supply the basal nuclei and internal capsule via a sinuous course: they are larger than the medullary branches and the longest of them reach close to the ventricle. The periventricular region and basal nuclei are also supplied by branches from the tela choroidea, which develops from the early pial plexus but becomes medially and deeply placed as the telencephalon enlarges.
The cortical and medullary branches irrigate a series of corticosubcortical cone-shaped areas, each centred around a sulcus containing an artery. They supply a peripheral portion of the cerebrum and are grouped as ventriculopetal arteries. In contrast, striate branches arborize close to the ventricle and supply a more central portion of the cerebrum; together with branches from the tela choroidea, they give rise to ventriculofugal arteries which supply the ventricular zone (germinal matrix of the brain) and send branches towards the cortex. The ventriculopetal and ventriculofugal arteries run towards each other but they do not make any connections or anastomoses: the ventriculopetal arteries form networks of small arterioles (Fig. 24.35). The ventriculopetal vessels supply relatively more mature regions of the brain compared to the ventriculofugal vessels, which are subject to constant remodelling and do not develop tunicae mediae until ventricular zone proliferation is completed. The boundary zone between these two systems (an outer centripetal and inner centrifugal) has practical implications related to the location of ischaemic lesions (periventricular leukomalacia, PVL) in the white matter of premature infant brains. Although it was thought that the distribution of ischaemic lesions in PVL coincided with the demarcation zone between the centrifugal and centripetal vascular arterial systems, this is now not thought to provide the complete answer. Three major interacting factors contribute to the pathology seen in PVL: the incomplete state of development of the vasculature in the ventricular zone, the maturation-dependent impairment of cerebral blood flow regulation in premature infants, and the vulnerability of oligodendroblasts in the periventricular region (which are particularly affected by swings in cerebral ischaemia and reperfusion) (Volpe 2001).
By 32–34 weeks, marked involution of the ventricular zone (germinal matrix) has occurred and the cortex acquires its complex gyral pattern and an increased vascular supply. Ventricular zone capillaries are gradually remodelled to blend with the capillaries of the caudate nucleus. Heubner’s artery eventually supplies only a small area at the medial aspect of the head of the caudate nucleus. In the cortex there is progressive elaboration of cortical blood vessels (Fig. 24.35) and towards the end of the third trimester the balance of cerebral circulation shifts from one which is central and basal nuclei-oriented, to one which predominantly serves the cortex and white matter. These changes in the pattern of cerebral circulation are of major significance in the pathogenesis and distribution of hypoxic/ischaemic lesions in the developing human brain. In a premature brain, the majority of ischaemic lesions occur in the boundary zone between the centripetal and centrifugal arteries, i.e. in the periventricular white matter, whereas in a full-term infant the cortical boundary zones and watershed areas between different arterial blood supplies are similar to those in adults and so presumably are the risks of ischaemic lesion.
VEINS
Veins of the head
The primary capillary plexus of the head is separated into three fairly distinct strata by the differentiation of the skull and meninges. The superficial vessels drain the skin and underlying soft parts, and eventually discharge in large part into the external jugular system, although they retain some connections with the deeper veins through so-called emissary veins. The next layer of vessels is the venous plexus of the dura mater, from which the dural venous sinuses differentiate: vessels from the plexus converge on each side into anterior, middle and posterior dural stems (Fig. 24.36). The anterior stem drains the prosencephalon and mesencephalon and enters the primary head vein rostral to the trigeminal ganglion. The middle stem drains the metencephalon and empties into the primary head vein caudal to the trigeminal ganglion, and the posterior stem drains the myelencephalon into the start of the precardinal vein. The deepest capillary stratum is the pial plexus from which the veins of the brain differentiate. It drains at the dorsolateral aspect of the neural tube into the adjacent dural venous plexus. The primary head vein also receives, at its cranial end, the primitive maxillary vein which drains the maxillary prominence and region of the optic vesicle.
The vessels of the dural plexus undergo profound changes, largely accommodating the growth of the cartilaginous otic capsule of the membranous labyrinth and the expansion of the cerebral hemispheres. As the otic capsule grows, the primary head vein is gradually reduced and a new channel joining anterior, middle and posterior dural stems appears dorsal to the cranial nerve ganglia and the capsule. The adult sigmoid sinus is formed where this channel joins the middle and posterior stems, together with the posterior dural stem itself (Fig. 24.36B).
Allen MC, Capute AJ. Tone and reflex development before term. J Pediatrics. 1990;85:393-399.
Provides details of the development of reflexes in extremely premature infants..
Begbie J, Graham A. The ectodermal placodes: a dysfunctional family. Phil Trans R Soc Lond B Biol Sci. 2001;356:1655-1660.
Brown M, Keynes R, Lumsden A. The Developing Brain. Oxford: Oxford University Press, 2001.
Covers the main mechanisms of neural development from neurulation to synaptic reorganization..
Bystron I, Blakemore C, Rakic P. Development of the human cerebral cortex: Boulder Committee revisited. Nat Rev Neurosci. 2008;9:110-122.
Donoghue MJ, Rakic P. Molecular gradients and compartments in the embryonic primate cerebral cortex. Cereb Cortex. 1999;9:586-600.
Gordon-Weeks PR. Neuronal Growth Cones. Cambridge: Cambridge University Press, 2000.
Kriegstein AR, Gotz M. Radial glia diversity: a matter of cell fate Glia. 2003;43(1):37-43.
Kriegstein AR, Noctor SC. Patterns of neuronal migration in the embryonic cortex. Trends Neurosci. 2004;27(7):392-399.
Krumlauf R, Marshall H, Studer M, Nonchev S, Sham MH, Lumsden A. Hox homeobox genes and regionalisation of the nervous system. J Neurobiol. 1993;24:1328-1340.
Lavezzi AM, Ottavianni G, Terni L, Matturri L. Histological and biological developmental characterization of the human cerebellar cortex. Int J Dev Neurosci. 2006;24:365-371.
Metin C, Baudoin JP, Rakic S, Parnavelas JG. Cell and molecular mechanisms involved in the migration of cortical interneurones. Eur J Neurosci. 2006;23(4):894-900.
Müller F, O’Rahilly R. The timing and sequence of appearance of neuromeres and their derivatives in staged human embryos. Acta Anat (Basel). 1997;158:83-99.
Noctor SC, Martinez-Cerdeno V, Ivic L, Kriegstein AR. Cortical neurones arise in symmetric and asymmetric division zones and migrate through specific phases. Nat Neurosci. 2004;7(2):136-144.
Opperman LA, Sweeney TM, Redmon J, Persing JA, Ogle RC. Tissue interactions with underlying dura mater inhibit osseous obliteration of developing cranial sutures. Dev Dynam. 1993;198:312-322.
Examines the role of the dura mater in the development of the skull bones and sutures..
O’Rahilly R, Müller F. The meninges in human development. J Neuropath Exp Neurol. 1986;45:588-608.
O’Rahilly R, Müller F. The Embryonic Human Brain. An Atlas of Developmental Stages. 2nd edition.. Chichester: Wiley-Liss; 1999.
O’Rahilly R, Müller F. The two sites of fusion of the neural folds and the two neuropores in the human embryo. Teratology. 2002;65:162-170.
O’Rahilly R, Müller F. The primitive streak, the caudal eminence and related structures in staged human embryos. Cells Tissues Organs. 2004;117:2-20.
O’Rourke NA, Sullivan DP, Kaznowski CE, Jacobs AA, McConnell SK. Tangential migration of neurones in the developing cerebral cortex. Development. 1995;121:2165-2176.
Rakic P. Specification of cerebral cortical areas. Science. 1988;241:170-176.
Rakic P. Developmental and evolutionary adaptations of cortical radial glia. Cereb Cortex. 2003;13:541-549.
The Boulder Committee. Embryonic vertebrate central nervous system: revised terminology. Anat Rec. 1970;166(2):257-261.
Storm EE, Garel S, Borello U, Hebert JM, Martinez S, McConnell SK, Martin GR, Rubenstein JL. Dose-dependent functions of Fgf8 in regulating telencephalic patterning centers. Development. 2006;133(9):1831-1844.
Volpe JJ. Neurobiology of periventricular leukomalacia in the premature infant. Pediatr Res. 2001;50:553-562.
von Melchner L, Pallas SL, Sur M. Visual behaviour mediated by retinal projections directed to the auditory pathway. Nature. 2000;404:820-821.
Withington S, Beddington R, Cooke J. Foregut endoderm is required at head process stage for anteriormost neural patterning in chick. Development. 2001;128:309-320.
Wonders CP, Anderson SA. The origin and specification of cortical interneurones. Nat Rev Neurosci. 2006;7(9):687-696.