CHAPTER 35 Development of the head and neck
Head development is distinct from that of the trunk, utilizing region-specific genes, signalling mechanisms and morphogenetic processes. This chapter will provide a description of head and neck development based primarily on data from studies on human embryos. Since this information is necessarily purely descriptive, it will be supplemented by observations from lineage and genetic studies in mouse embryos, where the results are likely to be valid for all mammals, including human. Human studies are taken from the following sources, which incorporate primary data by the authors as well as references to older primary source material: O’Rahilly and Müller (1987, 2001); Hinrichsen (1990). References to experimental studies in the mouse are provided in the text.
Evolution of the vertebrate head was made possible by the origin of a novel cell population, the neural crest. Neural crest cells have the potential to form connective and skeletal tissues in the head, and they make major contributions to the skull. In mammalian embryos, cranial neural crest cells emigrate from the edges of the still unfused cranial neural folds, unlike trunk neural crest cells and the cranial crest of other vertebrates, which begin migration only after neural tube closure. Figure 35.1 shows stylized views of human embryos at an early stage of neural crest migration (A) and at the end of the crest migration (B). N.B. These views do not show the neural crest cells themselves and not by a specific staining procedure.
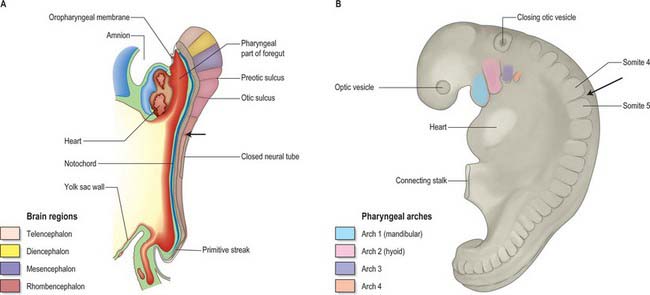
Fig. 35.1 Human embryos during early stages of head development. The occipital–cervical boundary is indicated by an arrow. A. Sagittal section of a stage 10 embryo in which neural tube closure has begun in the future occipito-cervical region. B. Lateral view of a stage 12 embryo; the occipital/cervical boundary between somites 4 and 5 is indicated by an arrow. (See also fig 24.2).
In the mouse, a lineage marker has enabled neural crest cells to be traced from the stage at which they leave the neural epithelium to their locations in adult tissues. These studies show three separate populations of neural crest cells migrating from the cranial neural folds which give rise to neuronal and non-neuronal progeny (Fig. 35.2). The first of these populations (trigeminal) originates from the diencephalic region of the forebrain, the midbrain, and prorhombomere A of the hindbrain, which subsequently divides to form the first two rhombomeres (Fig. 35.2A,B; see also Fig. 24.2). Those neural crest cells with a neuronal fate contribute to the trigeminal ganglion (Jiang et al 2002). The non-neuronal mesenchymal crest cells migrate extensively to surround the telencephalon and part of the diencephalon, forming the frontonasal mesenchymal populations; they also migrate lateral to the rhombencephalon to form the mesenchyme of the maxillary and mandibular regions of the first arch (Fig. 35.2C,D). The second (hyoid) population gives rise to the otic ganglion and then migrates from prorhombomere B (which forms rhombomeres 3 and 4), into the second pharyngeal arch. The third (vagal) population has a more extensive origin, from the neural folds caudal to the otocyst, i.e. prorhombomere C. These cells will contribute to the ganglia of the glossopharyngeal and vagal nerves, with the non-neuronal vagal crest cells migrating into pharyngeal arches 3, 4 and 6, some of them continuing into the heart to contribute to the division of the cardiac outflow tract. The neural crest also gives rise to the parasympathetic ganglia and parasympathetic postganglionic nerves in the head and neck. For a full account of the neuronal contribution of neural crest cells, see Chapter 24. In human embryos, histological methods have revealed equivalent cranial neural crest cell origins and migration routes to those of the mouse, except that no emigration from the diencephalon has been detected (O’Rahilly & Müller 2007).
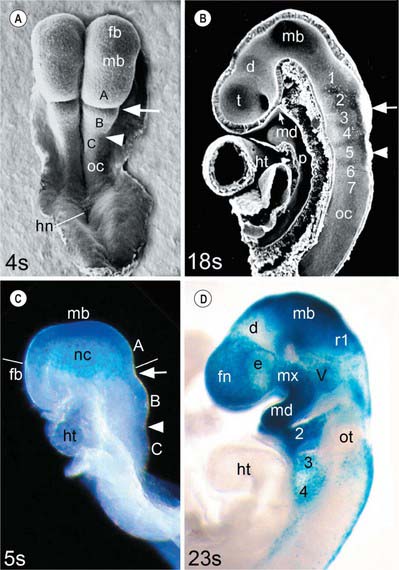
Fig. 35.2 Mouse embryo heads during the period of cranial neural crest cell migration. A and B, Scanning electron micrographs (A, dorsal view; B, median section) of embryos near the start (4 somite pairs) and end (18 somite pairs, interior view) of the period of neural crest cell migration. At the 4-somite stage the neural folds are convex and the hindbrain is divided into prorhombomeres A, B and C by the preotic (arrow) and otic (arrowhead) sulci; at the 18-somite stage the neural tube is closed and the hindbrain is divided into seven rhombomeres (numbered) and the unsegmented occipital region (oc). C and D, Lateral views of 5- and 23-somite stage embryos carrying a permanent marker for neural crest cells and the neural epithelium from which the trigeminal crest cells originate (blue stain). Neural crest cells (nc) migrate as a veil-like mass beneath the surface ectoderm, originating as a continuous group from prorhombomere A, the midbrain and the caudal diencephalic region of the forebrain, into the frontonasal region (fn) and the first arch (lines indicate the level of the migrating edge). After migration, frontonasal neural crest cells (fn) cover the telencephalon and rostral diencephalon, surrounding the eye (e); maxillary (mx) and mandibular (md) neural crest populates the first pharyngeal arch; the trigeminal ganglion primordium (V) is also present. Crest cells from prorhombomere B have populated the hyoid arch and the primordium of ganglion VII/VIII, rostral to the otocyst (ot); crest cells from prorhombomere C are migrating into arches 3 and 4 and starting to form the primordia of cranial ganglia IX and X, caudal to the otocyst. d, diencephalon; fb, forebrain; hn, Hensen’s node; ht, heart; mb, midbrain; oc, occipital region of neural fold/tube; p, pharynx; r1, rhombomere 1 of the hindbrain; t, telencephalon; Rathke’s pouch is indicated by a small arrow on B. (A and B, From Morriss-Kay and Wilkie 2005; C and D, from Jiang et al 2002.)
Caudal to the segmented region of the neural tube, neural crest cells from rhombomere 8 migrate with the occipital myotome-derived mesenchyme to form the hypoglossal cord, eventually differentiating to form the connective tissue (neural crest) and musculature of the tongue. No sensory ganglia are formed from the occipital neural crest in human embryos. The origin of the rhombomeres and brain regions in human embryos is given in Figures 24.2 and 24.12.
Ectodermal placodes also make important contributions to the developing head. There is good evidence to suggest that all cranial placodes originate from a continuous pre-placodal territory, the ectodermal ring, around the periphery of the early neural plate, distinct from neural and neural crest cell precursors; this territory is then subdivided into separate placodes, each with its distinct developmental fate (Bailey & Streit 2006). During neurulation the placodes are segregated into the surface ectoderm, moving within the epithelium to their final positions.
The first transverse division to form in the early embryonic brain is the preotic sulcus (Figs 35.1A, 35.2A), which defines the boundary between prorhombomeres A and B. This early division is of major functional and organizational significance, since it separates the regions of origin of the skeletogenic neural crest populations that make a major contribution to the skull from those that form skeletal structures in the neck (hyoid bone and larynx). During cranial neurulation the prorhombomeres undergo subdivision so that by the time the brain region of the neural tube closes, seven rhombomeres can be clearly distinguished; the occipital region (adjacent to the four occipital somites) remains unsegmented (Fig. 35.2B). By analogy with the segmented rhombencephalon, it is also referred to as prorhombomere D/rhombomere A. The segmental organization of the embryonic head caudal to prorhombomere A is related to the expression of evolutionarily conserved HOX genes (written Hox in the mouse) that have their rostral boundaries at specific rhombomere divisions; they are also expressed in the corresponding neural crest cells (reviewed by Santagati & Rijli 2003; see also Fig. 24.16). Genetic experiments in the mouse and other vertebrates have shown that skeletal patterning of the pharyngeal arch neural crest cells depends on the absence of Hox gene expression in the cells migrating into the first arch, and the expression of Hoxa2 in the second arch crest cells. Investigation of Hox gene functions in arches 3–6 has not revealed clear skeletal patterning functions.
EMBRYONIC PHARYNX AND PHARYNGEAL ARCHES
Each pharyngeal arch consists of an epithelial covering, ectoderm and endoderm, filled with mesenchyme which is mainly of neural crest origin, with some contribution from mesoderm (primitive streak-derived mesenchyme) (Fig. 35.3A,B; Table 35.1). The neural crest cells of each arch form a skeletal element and associated connective tissue; they also give rise to the walls of an aortic arch blood vessel which is lined with endothelium derived from angiogenic mesenchyme. Paraxial mesoderm forms the muscle associated with each pharyngeal arch and motor and sensory innervation derives from arch-specific cranial nerves. For an overview of the organization of these tissues in the pharynx, see Fig. 12.4; for their fate, see Figs 35.3, 35.5–35.8. Since the organization of the embryonic pharynx and related structures is similar in all vertebrate embryos, this phase has been termed ‘pharyngula’.
SKELETAL ELEMENTS OF THE PHARYNGEAL ARCHES
The skeletal elements of each arch are formed by condensation of neural crest-derived mesenchyme, which subsequently chondrifies in part or all of its length. Where chondrogenesis is complete (first and second arch cartilages), the element extends dorsally until it comes into contact with the cranial base lateral to the hindbrain (Fig. 35.5). An arch cartilage may remain as cartilage, undergo endochondral ossification, or become ligamentous, or a combination of these (Fig. 35.6).
The first arch cartilage, Meckel’s cartilage, forms the embryonic jaw skeleton. After the mandible forms by intramembranous ossification of the neural crest-derived mesenchyme lateral to it, Meckel’s cartilage degenerates but its sheath persists as the anterior malleolar and sphenomandibular ligaments. Its proximal end undergoes endochondral ossification to form two of the middle ear bones, the incus and malleus. These are homologous with the quadrate and articular bones of reptiles, and the joint between them is evolutionarily derived from the reptilian jaw articulation. In reptiles, the quadrate forms from the caudal end of the palatopterygoquadrate cartilage; the mammalian evolutionary derivatives of the palatopterygoquadrate are thought to include part of the greater wing of the sphenoid bone and the roots of its pterygoid plates, in addition to the incus. The portion of Meckel’s cartilage that extends from the mental foramen almost to the site of the future mandibular symphysis probably becomes ossified and incorporated into the intramembranous mandibular bone, and the remainder of the cartilage is ultimately absorbed.
MUSCLES OF THE PHARYNGEAL ARCHES
The striated muscle of each arch (sometimes termed branchiomeric) is derived from the rostral continuation of the paraxial mesoderm. The paraxial mesoderm of the head rostral to the occipital region is unsegmented, and the suggestion that a segmental pattern of seven cranial somitomeres exists is not generally accepted today. In the occipital region of the head, epithelialization takes place to form four pairs of somites; these are similar to those of the trunk. Myoblasts migrate from the paraxial mesoderm to sites of future muscle differentiation and form premuscle condensations prior to the development of any skeletal elements. The pattern of primary myotube alignment for any one muscle is specified by the surrounding neural crest-derived mesenchyme and is not related to the source of the myoblasts. The rate and pattern of muscle maturation are closely associated with the development of the skeletal elements but remain unattached until an appropriate time. Figs 35.3 and 35.7 show the muscle masses of each arch, their innervation and their derivatives in the adult.
The muscle mass of the mandibular part of the first arch forms tensor tympani, tensor veli palatini, mylohyoid, anterior belly of digastric, and the masticatory muscles (Fig. 35.7). Tensor tympani retains its connection with the skeletal element of the arch through its attachments to the malleus, and tensor veli palatini remains attached to the base of the medial pterygoid process – which may be derived from the dorsal cartilage of the first arch. However, the masticatory muscles become attached to the mandible, which is mainly a dermal bone. All of these muscles are supplied by the mandibular nerve, the mixed nerve of the first arch.
The muscles of the second arch migrate widely, but retain their original nerve supply from the facial nerve: migration is facilitated by the early obliteration of some of the first groove (cleft) and pouch. Stapedius, stylohyoid and posterior belly of digastric remain attached to the hyoid skeleton, but the facial musculature, platysma, auricular muscles and epicranius all lose connection with it (Fig. 35.7).
The muscle masses from arches 3 and 4 are adapted to form the musculature of the pharynx, larynx and soft palate. Stylopharyngeus is a third arch muscle, cricothyroid develops in the fourth arch (Fig. 35.7), and the rest of the laryngeal muscles are derived from the sixth arch. The precise origin of the remaining palatal muscles and the pharyngeal constrictors is uncertain. Palatoglossus, a muscle of the palate, is innervated by the cranial part of the accessory nerve and may derive from the lower arches.
NERVES OF THE PHARYNGEAL ARCHES
The nerves associated with each arch arise from the adjacent hindbrain (Fig. 35.7; see also Figs 12.4, 24.18). They are cranial nerves V (trigeminal), VII (facial), IX (glossopharyngeal), X (vagal) and XI (accessory). The motor nerves extend from the basal plate of the hindbrain to innervate the striated muscle of the arches: they are termed special branchial (visceral) efferent nerves because they innervate pharyngeal (branchiomeric) musculature. Sensory nerves extend peripherally and centrally from cranial ganglia that are formed partly from neural crest and partly from cells that delaminate from epipharyngeal placodes; they convey general and special somatic afferent axons. Each arch is innervated by a mixed nerve, but the nerves of arches 1–3 also have a purely sensory branch that innervates the arch rostral to its ‘own’ arch; this sensory nerve is called the pretrematic branch, because it extends rostral to the cleft or trema between the two arches (Figs 35.3, 35.7). Hence, the nerves to the mandibular arch include the mandibular division of the trigeminal nerve, which is mixed, and the chorda tympani and greater petrosal nerves, purely sensory pretrematic branches of the facial nerve. The maxillary branch of the trigeminal and the tympanic branch of the glossopharyngeal are also considered to be pretrematic nerves. The ophthalmic branch of the trigeminal nerve, which supplies the frontonasal area, is not an arch nerve; in fishes its ganglion is separate from the first arch nerve ganglion.
The vagus nerve, together with some fibres from the cranial part of the accessory nerve, supplies arches 3 (pharyngeal muscles), 4 and 6 (Fig. 35.7). The recurrent laryngeal branch initially loops under the sixth arch artery on both sides, but the asymmetric changes to the vascular system affect the symmetry of this nerve. On the right, where the sixth aortic arch disappears beyond the right pulmonary artery, the recurrent laryngeal nerve loops under the fourth arch-derived subclavian artery; on the left it loops round the ductus arteriosus (ligamentum arteriosum in the adult). Hence, the right and left branches of this nerve have asymmetric courses in the adult. Further details of cranial nerve development and composition are provided in Chapter 24.
BLOOD VESSELS OF THE PHARYNGEAL ARCHES
The aortic arches initially develop by vasculogenesis, soon after neural crest cells have invaded the early pharyngeal arches. Angiogenic mesenchyme forms the endothelial lining of the vessels and neural crest contributes to the outer layers of the walls (Jiang et al, 2000). The first aortic arch artery is part of the original vascular circuit that links the truncus arteriosus of the heart to the paired dorsal aortae, blood returning to the heart via the allantoic, vitelline and common cardinal veins (see Figs 13.1, 59.8). As the heart descends relative to the forebrain and other rostral structures, the aortic sac gives rise to paired aortic arches at successively more caudal levels, each of which passes laterally on each side of the pharynx to join the dorsal aortae. The dorsal aortae are not invested by neural crest cells, and nor are the more distal (cranial) parts of the carotid arteries, which form by angiogenesis. The whole complement of aortic arches never coexists – the first aortic arch degenerates through remodelling of the cranial arteries before the sixth is formed.
When the first and second aortic arch arteries begin to regress, the supply to the corresponding pharyngeal arches is derived from a transient ventral pharyngeal artery which terminates by dividing into mandibular and maxillary branches. The second aortic arch artery also degenerates, remaining only as the stem of the stapedial artery. The early vessel anastomoses with the ventral pharyngeal artery passing as it does through the mesenchyme of the stapedial cartilage, which condenses around it, forming the foramen of the stapes. The fully developed stapedial artery possesses three branches, mandibular, maxillary and supraorbital, which follow the divisions of the trigeminal nerve (Fig. 35.8). The mandibular and maxillary branches diverge from a common stem.
Further development of the third, fourth and sixth aortic arches (Fig. 35.9) produces the main arteries to the head and the great vessels arising from the heart. The external carotid artery first appears as a sprout, which extends cranially from the aortic sac close to the ventral end of the third arch artery. The common carotid arises from an elongation of the adjacent part of the aortic sac, and the third arch artery becomes the proximal part of the internal carotid artery. The fourth aortic arch on the right forms the proximal part of the right subclavian artery, whereas the corresponding vessel on the left constitutes the arch of the definitive aorta between the origins of the left common carotid and left subclavian arteries.
When the definitive ophthalmic artery differentiates as a branch from the terminal part of the internal carotid artery, it communicates with the supraorbital branch of the stapedial artery which distally becomes the lacrimal artery. The latter retains an anastomotic connection with the middle meningeal artery. The dorsal stem of the original second arch artery remains as one or more caroticotympanic branches of the internal carotid artery.
From its inception, the sixth aortic arch is associated with the developing lung buds. Initially, each bud is supplied by a capillary plexus from the aortic sac; as the sixth aortic arch develops, it becomes the channel for blood from the aortic sac to the developing lung buds as well as to the corresponding dorsal aorta, the latter being the main channel. Soon after formation of the sixth aortic arch, the outflow tract of the heart is divided by an influx of neural crest cells which form the spiral aorticopulmonary septum (Jiang et al 2000) which separates the aortic sac into the pulmonary trunk and ascending aorta; the pulmonary trunk attains alignment with the left sixth aortic arch (see p. 1025). The part of the sixth aortic arch between the pulmonary trunk and the dorsal aorta becomes the main vascular channel on the left, and is defined as the ductus arteriosus when the equivalent vessel on the right side is lost. Loss of the right sixth aortic arch may be related to the changes in blood flow dynamics consequent on division of the outflow tract. The caudal part of the right dorsal aorta (close to its conjunction with the left) also degenerates, separating the definitive aorta from the developing subclavian artery (Fig. 35.9B). The ductus arteriosus remains the main channel for blood to pass from the right ventricle to the descending aorta until the lungs and their associated blood vessels expand at birth. After birth, the ductus arteriosus is functionally closed by contraction of the circular muscle of the tunica media (see Ch. 59) (Fig. 35.9C).
PHARYNGEAL ECTODERM AND CLEFTS
Surface ectoderm lines the roof of the embryonic pharynx up to and including the adenohypophysial pouch (for the early development of the pituitary gland, see page 367 of Chapter 24). It also completely clothes the first arch covering the lateral walls and floor of the pharynx, unlike the more caudal arches which are dependent on the proximity of pharyngeal endoderm for their development. The external surface ectoderm of the first arch ultimately produces the keratinized stratified squamous epithelium of the epidermis including hair follicles, sweat and sebaceous glands, and the specialized epithelium of the vermilion of the lip. The first pharyngeal cleft is obliterated ventrally; its dorsal end deepens to form the external acoustic meatus and the external surface of the tympanic membrane (Fig. 35.4B,C); the ectoderm giving rise to the lining epidermis and ceruminous glands. The ectodermal covering of the oral aspect of the first arch forms the mucous membranes of the palate, oral cavity and anterior part of the tongue and the ectodermal components of the teeth, salivary and mucous glands. Three auricular hillocks form on arches 1 and 2, each side of the first pharyngeal groove beginning at stage 15; they form the auricle of the external ear (see Ch. 38).
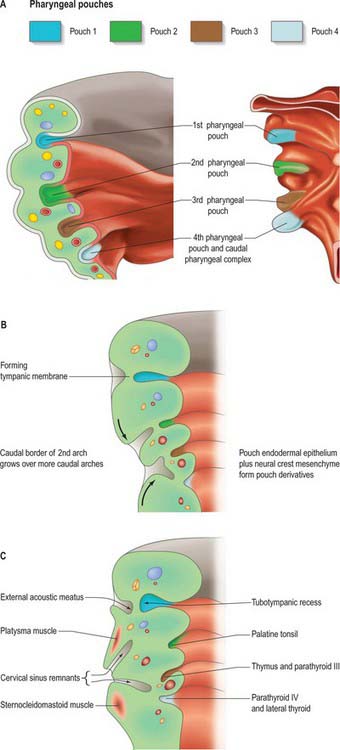
Fig. 35.4 Pharyngeal pouch development. A. The arrangement of the early pharyngeal pouches: on the left, the internal aspect of the pharyngeal floor viewed from above; on the right, the external aspect of the pharyngeal floor viewed from below. B. Coronal section of the left side of the pharynx at stage 18, showing changes to the pharyngeal pouches internally and pharyngeal clefts externally. C. Coronal section of the left side of the pharynx at stage 19. See also Fig 35.18 for further development.
The external contours of the arches and clefts are modified as the skeletal and muscular elements develop. During the fifth week, pharyngeal clefts 2 and 3+4 form the rostral and caudal parts of a retrohyoid depression, the cervical sinus (Fig. 35.4C). Cranially, the sinus is bounded by the hyoid arch; dorsally, by a ridge produced by ventral extensions from the occipital myotomes and by mesenchyme that develops into the sternocleidomastoid and trapezius muscles. Caudally, the smaller epipericardial ridge separates the sinus from the pericardium. Fusion of the hyoid arch with the cardiac elevation covers the cervical sinus, excluding the arches 3–6 from contributing to the skin of the neck; it also results in platysma muscle, bounded both superficially and deep with superficial fascia, passing along the neck to the anterior thoracic wall.
Thickened patches of ectoderm, the epibranchial placodes, appear at the dorsal ends of the pharyngeal grooves. They are closely related to the developing ganglia of the facial, glossopharyngeal and vagus nerves, to which they contribute. Together with dorsolateral and suprabranchial placodal cells, the epibranchial placodes also contribute to the trigeminal and vestibulocochlear ganglia (see Figs 12.4, 24.18).
PHARYNGEAL ENDODERM AND POUCHES
The first four pharyngeal pouches appear in sequence craniocaudally during stages 10–13. The rostral pharynx is broad and dorsoventrally compressed (see Fig. 35.18). Laterally, the endoderm of the pouches approaches the ectoderm of the pharyngeal clefts to form thin closing membranes (Fig. 35.4). The approximating ectoderm and endoderm between the first cleft and pouch form the outer and inner surfaces of the tympanic membrane. The ventral end of the first pouch is obliterated, but its dorsal end persists and expands as the head enlarges. This, together with the adjoining lateral part of the pharynx and possibly with a contribution from the dorsal part of the second pharyngeal pouch, constitutes the tubotympanic recess. The recess forms the middle ear cavity, the pharyngotympanic tube and their extensions. Expansion of the middle ear cavity around the ear ossicles occurs late during fetal life, by breakdown of the mesenchyme around the ossicles so that the cavity comes to surround them except at their attachments to the tympanic membrane and fenestra ovalis (fenestra vestibuli, oval window).
The ectoderm of the pharyngeal clefts and the endoderm of the pouches become increasingly separated by mesenchyme. The blind recesses of the second, third and fourth pouches are prolonged dorsally and ventrally as angular, wing-like diverticula (see Fig. 35.18); the pouch endoderm thickens and evaginates into localized neural crest-derived mesenchymal condensations. The ventral part of the second pouch is the focus of lymphoid development as the palatine tonsil. A generalized ring of lymphoid tissue, the lymphatic ring, develops in this region. The third pouch gives rise to the thymus ventrally and the parathyroid III dorsally, whereas the fourth pouch produces the parathyroid IV and an ultimobranchial body. The dorsal and ventral portions of the fourth pouch, together with the lower ultimobranchial body, are collectively termed the caudal pharyngeal complex.
FACE, NASAL CAVITIES AND PALATE, ORAL CAVITY AND TONGUE
FACE
By stage 12, migration of the most rostral neural crest cell population is complete and the sequence of morphogenetic changes that will form the face begins (Fig. 35.10). Neural crest cells have populated the mandibular arch and its dorsal maxillary extension, and formed the frontonasal mesenchyme that covers the telencephalon and caudal diencephalon. They also contribute to the primordium of the trigeminal nerve, whose first fibres develop at stage 13. The three divisions of the trigeminal nerve will provide sensory innervation to the frontonasal, maxillary and mandibular parts of the face. All of the mesenchyme of the facial region is neural crest-derived, except for the angiogenic mesenchyme, which has migrated from the cranial paraxial mesoderm to form the facial blood vessels. There are no muscle cells in this region during the morphogenetic period of facial development.
Localized mesenchymal proliferation results in the formation of four paired processes: the medial and lateral nasal, maxillary and mandibular processes. The lateral and medial nasal processes surround the nasal placodes, causing them to sink deep to the surface within the nasal pits. Below the developing nasal region, the median tissue forms the premaxillary component of the upper lip and jaw, and the primary palate. Concomitant with formation of the nasal processes, proliferation of the maxillary mesenchyme forms the maxillary processes. These make contact with the medial and lateral nasal processes at stage 16–17. Fusion of the maxillary processes with the medial nasal processes unites the premaxillary and maxillary parts of the upper jaw and lip; failure of this process on one or both sides causes unilateral or bilateral cleft lip (see Fig. 35.17). Superiorly, this fusion closes the cleft at the lower edge of the nasal pits, completing the future nostrils. Fusion of the maxillary with the lateral nasal processes is a much simpler process and rarely gives rise to abnormality. At stage 16, the epithelium of the groove between them thickens to form the lacrimal lamina, the primordium of the lacrimal system. At stage 19, it separates from the surface ectoderm, forming the lacrimal cord beneath the surface. The cord becomes canalized to form the nasolacrimal duct in the tenth week (de la Cuadra-Blanco et al 2006). The original site of the lacrimal lamina marks the lateral division between frontonasal and maxillary contributions to the face, the territories whose sensory nerve supply is respectively from the ophthalmic and maxillary divisions of the trigeminal nerve (Fig. 35.11).
The contribution made by the frontonasal process to surface epithelial derivatives extends from the skin of the forehead, over the supraorbital and glabellar regions including the upper eyelid and conjunctiva, to the external aspect of the nose and philtrum of the upper lip. During later development, the maxillary nerve invades the skin of the philtrum and nasal alae (Fig. 35.11; see Fig. 24.10). Internally, the epithelial contribution to mucous membrane includes the nasal vestibule, the nasal mucosa of the conchae and paranasal sinuses, the olfactory epithelium, the mucosa of the philtrum, gums and primary palate.
NASAL CAVITIES AND PALATE
Once the nasal cavities have formed, the roof of the oral cavity is regarded as the primitive palate (not to be confused with the primary palate). Each nasal cavity has an arcuate shape, extending upwards to the olfactory epithelium and then down again towards the oral cavity, from which it is separated by the thin epithelial choanal membrane. Towards the end of stage 18, the choanal membranes degenerate to form the choanae; the nasal cavites are now a duct from the external environment to the oral cavity. The triangular wedge of tissue between the left and right nasal cavities and the oral cavity comprises the primitive palate below and the nasal septum above. Caudal to the primary palate, the internal aspect of each maxillary process bulges into the oral cavity as a palatal process: the palatal processes (first detectable at stage 17) are the primordia of the secondary palate. The tongue is already well developed, and its domed form fills the space between the nascent palatal shelves laterally, and the primitive palate above. The mandible is at this stage short relative to the maxillary region and does not extend as far as the primary palate. Further growth brings the mandibular region and tongue forwards, to a position below the primary palate. At the same time, the (secondary) palatal processes continue to grow, projecting vertically downwards on either side of the tongue. At stage 23 (56–57 days), the tongue descends and the palatal shelves rotate, bringing the shelves to a horizontal position above the tongue (Fig. 35.12). The rapidity of the process of shelf elevation is caused at least in part by swelling of the palatal mesenchyme due to accumulation and hydration of hyaluronan (Ferguson 1991). This occurs during a period of continuous growth in head height but almost no growth in head width. This latter factor is important, because if palatal shelf elevation is delayed so that it occurs during a period of growth in facial width, the unfused processes may be unable to touch physically, resulting in a cleft palate. Other factors affecting palatal closure are the growth in length of Meckel’s cartilages, which allows the tongue to descend, and the change in position of the maxillae relative to the anterior cranial base, which has the effect of lifting the head and upper jaw away from the mandible during weeks 9 and 10, facilitating withdrawal of the tongue from between the palatal shelves and creating space for their elevation. Mouth opening, tongue protrusion and hiccup movements have also been noted at this time, and it may be that these movements and their associated pressure changes assist palatal shelf elevation. Generally, in female embryos palatal shelf elevation occurs 7 days later than in males, and congenital cleft palate is more common in females.
ORAL CAVITY
Tongue
Because the mandibular arch grows more rapidly than the others, this component of the pharyngeal floor is longer craniocaudally than that of the other arches. By stage 14, three swellings are apparent on its surface: a small median elevation, the tuberculum impar or median tongue bud, and paired mandibular (lingual) swellings. The mandibular swellings fuse with each other and with the tuberculum impar to form the anterior two-thirds of the tongue (Fig. 35.13). A sulcus forms along the ventral and lateral margins of this elevation and deepens, internal to the future alveolar process of the mandible, to form the linguogingival groove, while the elevation constitutes the anterior or oral (presulcal) part of the tongue. Caudal to the tuberculum impar, the copula and the hypobranchial (hypopharyngeal) eminence, form in the pharyngeal floor, and the ventral ends of the second, third and fourth pharyngeal arches converge onto them. The third arch component of the hypopharyngeal eminence grows over the second arch and approaches the anterior tongue rudiment; the two parts fuse along a V-shaped line corresponding later to the sulcus terminalis. Another transverse groove separates its caudal (fourth arch) part to delineate the epiglottis.
During this sequence of events, the second arch is excluded from the tongue. The sulcus terminalis has its apex at the foramen caecum: this is a small median diverticulum that forms at the time of fusion of the constituent parts of the tongue, and is the site of downgrowth of the median rudiment of the thyroid gland. Failure of downgrowth of all or part of the thyroid gland from this site results in ectopic thyroid tissue within the tongue (lingual thyroid), between the foramen caecum and the epiglottis. The tongue primordia fuse at stage 17 (15 mm), and the sulcus terminalis is formed by the line of fusion. The vallate papillae appear around 10 weeks (52 mm), and increase in number until the end of the second trimester. Serial reconstructions suggest that the territory of the glossopharyngeal nerve extends considerably beyond these papillae. However, in general the composite character of the mucous membrane of the tongue is reflected by its sensory innervation. The anterior, oral part is innervated by the lingual branch of the mandibular nerve, and by the chorda tympani of the facial nerve. The posterior, pharyngeal part of the tongue is innervated by the glossopharyngeal, the nerve of the third arch, and the root of the tongue, near the epiglottis, is innervated by the vagus. During stages 14 and 15, the mesenchyme of the tongue is invaded by cells from the occipital myotomes which migrate from the lateral aspects of the myelencephalon. They pass ventrally round the pharynx to reach its floor accompanied by the hypoglossal nerves. The posterior parts of the tongue and epiglottis, i.e. the embryonic pharyngeal floor derivatives of the third, fourth and sixth arches, lie within the oropharynx. Striations appear on the surface of the tongue early in the fetal period. The tongue is short and broad, and its entire surface lies within the oral cavity (see Fig. 14.5). The posterior third of the tongue descends as the hyoid bone and larynx descend during the first postnatal year, and by the fourth or fifth year it forms part of the anterior wall of the oropharynx.
Lymphoid tissues
Tonsils form at several sites around the oral cavity and pharynx, where focal proliferations of endoderm become invaded by lymphoid tissue. The endodermal epithelial lining grows into the surrounding mesenchyme as a number of solid buds, which are excavated by degeneration and shedding of their central cells, forming tonsillar fossae and crypts. Lymphoid cells accumulate around the crypts and become grouped as lymphoid follicles; T- and B-cell regions can be identified. The palatine tonsils develop from the ventral parts of the second pharyngeal pouches (Fig. 35.4; see Fig. 35.18). A slit-like intratonsillar cleft extends into the upper part of the tonsil and is possibly a remnant of the second pharyngeal pouch. Lymphoid tissue similar to that of the palatine tonsils usually develops on the surface of the posterior part of the tongue, forming the lingual tonsil.
Teeth and gums
Demarcation of the lips begins after fusion of the facial primordia at stage 18, during the period of secondary palatal development. Two parallel epithelial thickenings, an outer labiogingival lamina (vestibular band) and an inner dental lamina, form around the interior border of the mouth in both upper and lower jaws (Fig. 35.13; see Fig. 35.15). The linguogingival groove (sulcus) is formed as an indentation between the dental lamina and the tongue. At stage 22, each labiogingival lamina indents the underlying mesenchyme to form a shallow groove that deepens to form the vestibule between the lips and gums. The lining of the oral cavity between the vestibular epithelium of the upper and lower jaws forms the inner aspect of the cheeks. The dental lamina gives rise to the epithelial component of the teeth.
Tooth formation involves a sequence of epithelial–mesenchymal interactions along the dental lamina (Fig. 35.14). These interactions are controlled by a large number of genetic factors, and the extracellular matrix (particularly of the basement membranes) is of major importance (Thesleff 2000, Fukumoto & Yamada 2005). In 25 mm embryos, 10 localized thickenings of the upper and lower dental laminae initiate formation of the dental epithelial components of the tooth primordia (Figs 35.14, 35.15). They expand into dental sacs surrounded by vascular mesenchyme. The epithelium proliferates and indents to form an enamel organ which by 10 weeks forms a cap over a mesenchymal condensation, the dental papilla: collectively this unit constitutes a tooth bud or germ. The enamel organ becomes bell-shaped and differentiates into two layers, the internal and external enamel epithelia; these are separated by a glycosaminoglycan-rich stellate reticulum. The cells of the internal epithelium differentiate into ameloblasts, and the underlying layer of mesenchymal cells differentiates into odontoblasts. The odontoblasts produce and secrete dentine, which influences the ameloblasts to form enamel; this is laid down in successive layers, and after mineralization is the hardest tissue in the body. Enamel and dentine production from the bell-shaped tooth germ both begin at 24 weeks. The dental papilla mesenchyme below the odontoblast layer forms the pulp, which becomes vascularized and innervated. Experimental studies in mice (which have only incisors and molars) have revealed that regional differences in gene expression within the maxillary and mandibular mesenchymes govern the type of tooth produced. Fibronectin is present in the basement membrane of the internal enamel epithelium during the bell phase; it assists attachment of the preodontoblasts and is essential for their differentiation; it becomes progressively more abundant during the later fetal period. Collagen I is the major supportive extracellular scaffold in the maturing dental papilla and stellate reticulum.
The oral cavity after birth
In the neonate, the oral cavity is only potential with the mouth closed. Three spaces are formed in the oral cavity during suckling. The median space between the tongue and hard palate that is occupied by the nipple bifurcates posteriorly to produce channels on each side of the approximated soft palate and epiglottis. The hard palate is only slightly arched and its mucous membrane is corrugated by five or six irregular transverse folds (rugae) which assist with gripping of the nipple. Two lateral spaces, the lateral arcuate cavities, are formed between the tongue medially and the cheeks laterally; the upper and lower gums situated within these spaces do not touch during suckling. Each cheek is supported by a mass of subcutaneous fat, the suctorial pad, which lies between buccinator and masseter. The epiglottis is high and makes direct contact with the soft palate (see Fig. 14.15). The larynx is elevated so that its opening is directed into the nasopharynx, i.e. above the level of the spaces, as milk passes on either side of it to the pharynx. This ensures that babies can breathe while suckling.
ANOMALIES OF FACIAL DEVELOPMENT
Malformations involving the neural crest
Congenital malformations of the face may be initiated early, and related to abnormal migration, proliferation and/or apoptosis of neural crest cells, or later, during the morphogenetic phase of facial development. Craniofrontonasal syndrome is characterized by a broad forehead and hypertelorism, premature fusion of one or both coronal sutures, a central nasal groove, and sometimes cleft lip and palate; there are also extracranial features. It is caused by deletions of the gene EFNB1, which is known to play essential roles in boundary formation between tissues, including those between neural crest and adjacent non-crest cells. Mandibulofacial dysostosis (Treacher Collins syndrome) is characterized by incomplete orbits, reduced jaws and auditory ossicles (hence conductive deafness), abnormal external ears and fistulae or cysts, suggesting involvement of all levels of the cranial neural crest. It is caused by haploinsufficiency of the gene TCOF1. Hemifacial microsomia (Goldenhaar syndrome) affects only one side of the face and comprises a small mandible (sometimes with absence of the temporomandibular joint), malformed or absent ears with accessory ear tags, and facial clefts. Experimental evidence in animals suggests that it is due to haemorrhage of the stapedial artery, which may have a genetic basis. Deficient growth of the mandible (micrognathia) is usually associated with other defects. In Pierre Robin sequence (Fig. 35.16), the small size of the mandible obstructs descent of the tongue, resulting in a U-shaped cleft palate; a conotruncal septum defect is present in one-quarter of cases, suggesting possible involvement of the cardiac neural crest. Further details and references for facial malformations may be found in Gorlin et al (2001), Wilkie and Morriss-Kay (2001), and Morriss-Kay and Wilkie (2005).
Clefts of the face and palate
Cleft lip occurs when one or both medial nasal processes fail to fuse with the corresponding maxillary process(es). The severity of clefting varies from a small notch in the upper lip to a double cleft extending into both nostrils (Fig. 35.17). In a unilateral cleft lip, the nasal septum deviates to the non-cleft side due to absence of the correct insertion of the transverse muscles of the nose and of orbicularis oris on the medial aspect of the cleft onto the tissues around the anterior nasal spine and nasal septum and, most importantly, to the contralateral muscles (Fig. 35.17B). The three functional groups of superficial facial muscles – nasolabial (transverse nasalis, levator labii superioris and levator labii superioris alequae nasi), bilabial (orbicularis oris) and labiomental (depressor anguli oris) – are all displaced inferiorly. A further consequence is the underdevelopment of the incisor-bearing part of the maxilla. These abnormalities, in turn, influence the mucocutaneous tissues, which results in the displacement of the skin of the nostril to the upper part of the lip, retraction of the labial skin and abnormalities of the soft tissues on either side of the mucocutaneous junction.
A narrow or incomplete cleft of the hard palate is likely to be due to a growth defect in the palatal shelves and/or a delay in shelf elevation, and/or to failure of fusion of the apposed palatal shelves. Broader clefts (Fig. 35.17D,E), in which the tongue lies between the still-vertical shelves, are caused by a greater developmental delay that may be associated with abnormality of other structures in the face and elsewhere in the body. In the mildest forms of cleft palate, only the soft palate, and sometimes just the uvula, is cleft (Fig. 35.17F). A submucous cleft can cause speech difficulties; it may be detected as a median V-shaped notch of the soft palate. Very rarely, palatopharyngeal incompetence is due to muscle hypoplasia, particularly of the musculus uvulae.
Cleft lip with or without cleft palate (CL/P) appears to be multigenic or multifactorial in origin, with both genetic and environmental components (Gorlin et al 2001). CL/P has a frequency of 0.5–3.6/1000 live births, with a 2 : 1 male : female ratio. Isolated cleft palate is genetically unrelated to CL/P, and has a frequency of 0.4/1000; it is more common in females. Clefts of the face are rare (1/50,000–1/175,000 births); they are mainly caused by pressure from amniotic bands rather than from dysmorphogenesis, although a morphogenetic component cannot be ruled out when the cleft is along a line of fusion, e.g. maxillary–mandibular. Median cleft lip (true hare lip) is also rare, since this is not a line of morphogenetic fusion. Median grooving of the nose occurs with hypertelorism in various frontonasal dysplasias, suggesting an early broadening of the median region of the face.
FORMATION OF THE NECK
The interface between cranial nerve-innervated pharyngeal arch muscles and spinal nerve-innervated trunk muscles occurs at the lower neck and the upper parts of the pectoral girdle. Both the proximal and distal connections of the trapezius muscle, at the nuchal line of the occipital bone and the attachment to the spine of the scapula, are formed from post-otic neural crest cells (Matsuoka et al 2005). The neural crest generates areas of endochondral ossification in the cervical vertebral column and scapula associated with trapezius attachment. This observation has promoted the conception of a ‘muscle scaffold model’ of development to replace the older ‘ossification model’, to explain the types of ossification seen associated with the accessory nerve-innervated trapezius and sternocleidomastoid muscles. It is suggested that defects in the fate choices of neural crest cells could explain a number of syndromes which have pharyngeal, laryngeal, occipital, cervical and shoulder dysmorphologies and co-occurrence of swallowing problems; related syndromes include Klippel–Feil, Sprengel’s deformity and cleidocranial dysplasia (Matsuoka et al 2005).
GLANDS OF THE NECK
Thyroid gland
The thyroid gland is a midline derivative of the pharynx. It is first identifiable in embryos of approximately 20 somites as a median thickening of endoderm lying in the floor of the pharynx between the first and second pharyngeal pouches and immediately dorsal to the aortic sac. This area is later invaginated to form a median diverticulum which appears late in the fourth week in the furrow immediately caudal to the median tongue bud (Fig. 35.18). It grows caudally as a tubular duct. The tip of the duct bifurcates and the tissue mass subsequently divides into a series of double cellular plates, from which the isthmus and the lateral lobes of the thyroid gland are developed. The primary thyroid follicles differentiate by reorganization and proliferation of the cells of these plates. Secondary follicles subsequently arise by budding and subdivision. These primary and secondary endodermal cells are the progenitors of the follicular parenchyma proper. The parafollicular or C cells of the thyroid gland are derived from the ultimobranchial body.
The thyroid gland is relatively large in the neonate (see Fig. 14.4), where it has a long narrow isthmus connecting lobes which do not yet contact the upper part of the trachea. The gland attains half the adult size by 2 years postnatally. Colloid is present in the gland from 3 months’ gestation and thyroxin is present by 4.5 months’ gestation.
Parathyroid glands
The parathyroid glands develop from interactions between the third and fourth pharyngeal pouch endoderm and local cranial (vagal) neural crest mesenchyme. The third pharyngeal pouch has dorsal and ventral sites of proliferation (Fig. 35.18). Bilaterally, the epithelium on the dorsal aspect of the pouch and in the region of its duct-like connection with the cavity of the pharynx becomes differentiated as the primordium of the inferior parathyroid glands (parathyroid III). Although the connection between the pouches and the pharynx is soon lost, the connection between the dorsal parathyroids III and the ventral thymic rudiments persists for some time, and the former passes caudally with the developing thymus. The superior parathyroid glands (parathyroid IV) develop from the dorsal recess of the fourth pharyngeal pouches. They come into relation with, and appear to be anchored by, the lateral lobes of the thyroid gland and thus remain cranial to the parathyroid glands derived from the third pouches. The cardiac neural crest mesenchyme provides the connective tissue elements, whereas invading angiogenic mesenchyme gives rise to the vasculature which includes fenestrated capillaries and lymphatics. In the neonate the parathyroid glands are as variable in size and position as they are in the adult. They double in size between birth and puberty. Parathyroid hormone is produced from the 12th week of development.
Thymus
The thymus gland is formed from the ventral part of the third pharyngeal pouch on each side (Fig. 35.18). It cannot be recognized prior to the differentiation of the inferior parathyroid glands at stage 16, but thereafter it is represented by two elongated diverticula which soon become solid cellular masses and grow caudally into the surrounding cardiac (vagal) neural crest mesenchyme. Ventral to the aortic sac the two thymic rudiments meet but do not fuse, and they are subsequently united by connective tissue only. The thymus gland becomes located in the anterior mediastinum of the thorax once the neck is fully developed and the heart has descended. The connection with the third pouch is soon lost, but the stalk may persist for some time as a solid, cellular cord.
Caudal pharyngeal complex
The most caudal endodermal invaginations of the pharynx are the fourth pharyngeal pouch and elements of the transitory fifth pharyngeal pouch (the ultimobranchial body). Collectively these diverticuli are termed the caudal pharyngeal complex (Fig. 35.18), and they are connected to the pharynx via the pharyngobranchial duct. They are surrounded by cardiac (vagal) neural crest and by the tissues of the developing thyroid gland. The cells of the ultimobranchial body, the lowest of the pharyngeal pouches, become incorporated into the lateral thyroid lobes, and may give rise to the ‘C’ or parafollicular cells of the thyroid gland. C-cell hyperplasia is associated with medullary carcinoma, and has been reported within the neck in what are presumed to be remnants of the ultimobranchial body.
PHARYNX, LARYNX, OESOPHAGUS AND TRACHEA
The larynx forms at the cranial end of the laryngotracheal groove, where it is bounded laterally by the ventral ends of the sixth arches and ventrally by the caudal part of the hypobranchial eminence (Figs 35.5, 35.6, 35.13). The thyroid cartilage develops from the ventral ends of the cartilages of the fourth, or fourth and sixth, pharyngeal arches. The cartilage appears as two lateral plates, each chondrified from two centres and united in the midventral line by a fibrous membrane within which an additional centre of chondrification develops. The cricoid cartilage arises from two cartilaginous centres, which soon unite ventrally, gradually extend, and ultimately fuse on the dorsal surface of the tube as the cricoid lamina. Paired arytenoid swellings appear in the ventral ends of the sixth arches from stage 14, one on each side of the cranial end of the groove. As they enlarge, they approximate to each other and to the caudal part of the hypobranchial eminence where the epiglottis develops. The opening into the larynx, at first a vertical slit, is converted into a T-shaped cleft by the enlargement of the arytenoid swellings. The vertical limb of the T lies between the two swellings, and its horizontal limb lies between them and the epiglottis. The arytenoid swellings differentiate into the arytenoid and corniculate cartilages (Fig. 35.6), and the ridges that join them to the epiglottis become the definitive aryepiglottic folds within which the cuneiform cartilages differentiate from the epiglottis. After the caudal part of the hypobranchial eminence has separated from the pharyngeal (posterior) part of the tongue (Fig. 35.13), it is in continuity with two linear ridges which appear in the ventral wall of the pharynx. The ridges are the sixth arches, which are placed very obliquely owing to the shortness of the pharyngeal floor compared with the greater extent of the roof. They are carried downwards on the ventral wall of the foregut and bound the median laryngotracheal groove, from which the lower part of the larynx, the trachea, bronchi and lungs are developed (see Ch. 59).
From stage 12, the early pharyngeal foregut develops a ventral endodermal outgrowth into the mesenchyme surrounding the sinus venosus and inflow tract of the heart (see Figs 59.1, 73.3). This initiates the separation of the respiratory and alimentary tubes. The point at which the original respiratory diverticulum buds from the foregut, the laryngotracheal groove, remains at a constant level during the embryonic period, and the trachea lengthens distally as the bifurcation point descends. The cells of the splanchnopleuric mesenchyme that surrounds the developing trachea and oesophagus are sufficiently diverged in their inductive ability to promote the characteristics of the two different tubes. The respiratory diverticulum becomes surrounded by angiogenic mesenchyme that connects to the developing sixth aortic arch artery. By stage 17, the mesenchyme around the trachea is beginning to condense to form C-shaped cartilages, whereas that around the oesophagus has a wide submucosal zone and muscular coats can be distinguished.
For details of early development of the trachea see p. 1033, and early development of the oesophagus see p. 1203.
In the normal neonate the hyoid bone and larynx are relatively high in the neck; both descend during infancy, and the trachea is relatively small in relation to the larynx (see Fig. 14.4). The walls of the trachea are relatively thick and the tracheal cartilages are relatively closer together than in the adult. The trachea commences at the upper border of the sixth cervical vertebra, a relationship that is conserved with growth, and it bifurcates at the level of the third or fourth thoracic vertebra.
BLOOD VESSELS IN THE NECK
With elongation of the neck and the appearance of the major conducting vessels from the aortic arch arteries, longitudinal anastomoses in the cervical region link intersegmental arteries and their branches and direct blood flow to the developing brain in the vertebral arteries, in parallel with the internal carotid arteries (see Figs 35.8, 35.9).
The primary blood vessels of the head and neck consist of a close-meshed capillary plexus drained on each side by the precardinal vein, which is at first continuous cranially with a transitory primordial hindbrain channel that lies on the neural tube medial to the cranial nerve roots (see Figs 13.1, 13.2). This is soon replaced by the primary head vein which runs caudally from the medial side of the trigeminal ganglion, lateral to the facial and vestibulocochlear nerves and the otocyst, then medial to the vagus nerve, to become continuous with the precardinal vein. A lateral anastomosis subsequently brings it lateral to the vagus nerve.
The ventral pharyngeal vein drains the mandibular and hyoid arches into the common cardinal vein (see Fig. 24.36A). As the neck elongates, its termination is transferred to the cranial part of the precardinal vein which later becomes the internal jugular vein (see Fig. 24.36B). The ventral pharyngeal vein receives tributaries from the face and tongue and becomes the linguofacial vein. As the face develops, the primitive maxillary vein extends its drainage into the territories of supply of the ophthalmic and mandibular divisions of the trigeminal nerve, including the pterygoid and temporal muscles, and it anastomoses with the linguofacial vein over the lower jaw. This anastomosis becomes the facial vein which receives a strong retromandibular vein from the temporal region, and drains through the linguofacial vein into the internal jugular. The stem of the linguofacial vein is now the lower part of the facial vein, whilst the dwindling connection of the facial with the primitive maxillary becomes the deep facial vein. The external jugular vein develops from a tributary of the cephalic vein from the tissues of the neck; it anastomoses secondarily with the anterior facial vein. At this stage the cephalic vein forms a venous ring around the clavicle by which it is connected with the caudal part of the precardinal vein. The deep segment of the venous ring forms the subclavian vein and receives the definitive external jugular vein. The superficial segment of the venous ring dwindles, but may persist in adult life. The deep aspects of the maxillomandibular facial prominences, retrogingival oral cavity, the pharyngeal walls and their lymphoid and endocrine derivatives, and the cervicothoracic oesophagus, thus all have drainage channels that connect with the precardinal complex. Laryngeal and tracheobronchial veins also drain to the precardinal complex, whilst the capillary plexuses, developed in the (splanchnopleuric) walls of the fine terminal respiratory passages and alveoli, converge on pulmonary veins of increasing calibre, finally making secondary connections with the left atrium of the heart, and may be grouped with the vitelline systems.
SKULL
The skull has two major functional and anatomical components: the neurocranium and viscerocranium. The neurocranium is composed of the skull vault and skull base; it surrounds and protects the brain and the special sense organs of olfaction, vision, hearing and balance. The viscerocranium forms the skeleton of the face, palate and pharynx, and mediates the functions of feeding, breathing and facial expression; it also protects the tongue and forms the middle ear and the bony external acoustic meatus. The most rostral neural crest cell population (see introductory section) makes a major contribution to the skull, forming the whole viscerocranium and the rostral part of the neurocranium (Fig. 35.19). The boundary between neural crest and cranial mesoderm lies between the frontal and parietal bones (coronal suture) of the skull vault; the skull base is formed by neural crest rostral to the tip of the notochord, and is sclerotome-derived (i.e. mesodermal) in the notochordal region. The bones of the skull base are formed by endochondral ossification, whereas those of the skull vault and face ossify directly from mesenchymal condensations (intramembranous ossification). Several bones are of compound structure with respect to their tissue origins and/or type of ossification: the occipital, temporal and sphenoid bones, and the mandible. The following account is based largely on descriptive studies on human embryos and fetuses (O’Rahilly & Müller 1987, 2001, 2007), with some details on cell lineage based on investigations in the mouse.
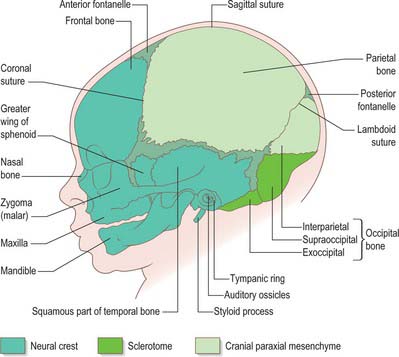
Fig. 35.19 Newborn skull, showing tissue origins of the bones (based on combined mouse and human data).
CHONDROCRANIUM
The first cranial skeletal structures to differentiate are the cartilages of the skull base, sensory capsules, viscerocranium and occiput (Figs 35.5, 35.20B). Development of the pharyngeal arch-related cartilages of the viscerocranium and ear ossicles has been described in the pharynx section above. Before any mesenchyme condenses to initiate the formation of cranial cartilage, the major cranial nerves and blood vessels are already in place – hence, the foramina of the skull are specified before the bones are formed. The adenohypophysial pouch is still connected to the roof of the oral cavity as the skull base cartilage forms, but already lies adjacent to the diencephalon-derived neurohypophysis to form the downwardly projecting pituitary gland (hypophysis) at the base of the caudal diencephalon.
The rostral tip of the notochord extends to a position just caudal to the hypophysis (Fig. 35.20A). Its sheath is rich in sulphated glycosaminoglycans, which play a role in inducing condensation and chondrification of the occipital sclerotome-derived mesenchyme on either side of it.
The otic capsule differentiates from a mesenchymal condensation around the otocyst, after its morphogenesis to form the cochlea and semicircular canals. Differentiation of cartilage begins laterally, at the same time as the polar cartilages are first detectable; it is complete by stage 20. Chondrogenesis around the point of exit of the vestibulocochlear nerve creates the internal acoustic meatus. Chondrogenesis of mesenchyme around the carotid arteries joins each polar cartilage to the otic capsule, forming the carotid canals. A gap occupied by the jugular vein remains between each otic capsule and the parachordal cartilage: this is the jugular foramen. Chondrogenesis of the mesenchyme between the separate cartilages completes formation of the cartilaginous skull base and sensory capsules, which together form a continuous framework around the pre-existing blood vessels and cranial nerves by 9 weeks (40 mm) (Fig. 35.20B).
SKULL VAULT
The bones of the skull vault or calvaria (roof and lateral walls of the neurocranium) are formed entirely by intramembranous ossification. They are also described as dermal bones, since they are considered to be the evolutionary descendants of dermal plates formed as a protective cover for the brain in fishes. In humans, as in all mammals, the major part of the skull vault is formed by paired frontal and parietal bones and the unpaired interparietal (membranous part of the occipital bone). The squamous part of the temporal bones and the membranous part of the alisphenoid contribute to the lateral walls. Lineage data from mouse studies indicates that the frontal and squamous temporal bones are of neural crest origin and the parietals are of mesodermal origin; the interparietal is mixed (Jiang et al 2002). The coronal suture thus forms at the neural crest–mesoderm interface, as does the sagittal suture, due to a small tongue of neural crest tissue lying between the two developing bones. These tissue interfaces may be significant for initiating the molecular signalling system that governs growth of the skull vault. Growth at the borders of adjacent bone, i.e. in the sutures, is the major mechanism of skull vault growth. A sequence of events that maintains a balance between cell proliferation and osteogenic differentiation is mediated by an intercellular signalling system that includes the transcription factor TWIST and ligand–receptor interactions between fibroblast growth factors (FGFs) and their receptors (FGFRs). Mutations in the genes encoding these proteins cause premature fusion of the cranial sutures (craniosynostosis) (for further details and references for this and related descriptions in this section, see Morriss-Kay & Wilkie 2005). In addition to the expansion-related growth that takes place in the sutures, appositional growth, in which bone is laid down on, and resorbed from, the bone surfaces, plays an important role in remodelling the calvarial bones to maintain a degree of curvature that matches the curved surface of the growing brain.
The frontal and parietal bones are initiated as basolateral mesenchymal primordia which extend upwards between the dermal connective tissue and the mesenchymal dura mater. They do not, as previously thought, differentiate within the mesenchyme of an ‘ectomeninx’ that surrounds the brain. As the frontal and then the parietal primordia extend upwards, the differentiating osteoblasts secrete osteoid which then undergoes mineralization. Only the mineralized parts of the bones are detected in Alizarin-stained specimens and X-rays. Clear frontal bone primordia are detectable in the superciliary arch region of Alizarin-stained human embryos by the eighth week. As they become mineralized above this level, the parietal bone primordia can also be seen. The two bones appear to be separated by a wide gap at the coronal suture; in fact, this gap only shows the separation of the mineralized parts of the bone – the unmineralized edge of the parietal bone actually overlaps the caudal edge of the frontal bone. After the upgrowth phase, mineralization takes place centrifugally from the central points of the frontal and parietal bones, which by this time form convex plates over the curvature of the underlying brain.
The interparietal bone (supranuchal squamous portion of the occipital bone) forms from two ossification centres that appear in the eighth week. These are considered to be homologous with the paired postparietal bones of reptiles. The fact that they unite to form a single bone in mammals may be due to the interpolation of a small area of neural crest that migrates from the hindbrain after neural tube closure (Jiang et al 2002). Two further ossification centres develop laterally at 12 weeks. There is at first a wide gap occupied by cartilage between the interparietal and parietal bones, which disappears as the membrane bones grow towards each other, forming the lambdoid suture when they abut. The cartilage is in a deeper tissue layer than the intramembranous bone, reflecting the dermal origins of the latter. The lambdoid suture contributes growth to the caudal border of the parietal bones and to the upper part of the occipital bone. Synostosis of this suture is relatively rare (0–5% of craniosynostosis cases) and has a less severe effect on overall skull growth than coronal and median fusions. Posteriorly, between the interparietal bone and the foramen magnum, the skull vault is not formed by membrane bones but by endochondral ossification of the supraoccipital component of the occipital bone.
MEMBRANE BONES OF THE FACE AND VISCEROCRANIUM
During migration, the trigeminal neural crest cells divide into a frontonasal population that migrates superior to the eye, a mandibular population that migrates into the first pharyngeal arch, and a maxillary population closely associated with the proximal end of the first arch (Fig. 35.2). The frontal, lacrimal, nasal bones, the vomer and the premaxillary (incisor tooth-bearing) part of the maxilla are derived from the frontonasal mesenchyme; the maxilla and zygoma are derived from the maxillary mesenchyme; the mandible and tympanic bone are derived from the mandibular mesenchyme. The mandible is the first membrane bone to begin ossification: its single ossification centre appears in the seventh week. The maxilla and premaxilla have primary ossification centres by 7 weeks, and three further ossification centres form in the maxillary mesenchyme at 8 weeks; all of these components fuse to form a single bone, in contrast to some mammals in which the premaxilla remains separate. In the neonatal skull, the suture between the primary and secondary parts of the palate is still patent, indicating the premaxillary contribution here. By 8 embryonic weeks, ossification centres for most of the facial and viscerocranial bones are present, except for the tympanic ring, for which four ossification centres appear at 12 weeks. They fuse to form the sickle-shaped bone that supports the tympanic membrane.
POSTNATAL GROWTH OF THE SKULL
Postnatal growth of the skull is characterized by changing proportions of its components. Growth of the brain continues to be extremely rapid in the first 2 years; in addition to continuing growth of the frontal and parietal bones, the squamous temporal bone increases in size so that it contributes a greater proportion of the skull vault in the adult than in the neonate (Fig. 35.21). The inner ear and the petrous temporal bone around it grow very little after birth, so the increasing breadth of the skull draws the petrous temporal bone out laterally, creating the bony external acoustic meatus. The tympanic ring (with the tympanic membrane) lies at the surface of the meatus in the neonatal skull; it remains at the proximal end of the deepening canal. Use of the sternocleidomastoid muscle to lift the head results in formation of the mastoid process of the temporal bone, which develops air-filled spaces that are continuous with the middle ear cavity. The paranasal sinuses begin to form in late fetal life as diverticula from the nasal cavity which gradually invade the maxilla, frontal, ethmoid and sphenoid bones. At birth they fill with air when the amniotic fluid drains from them. Thickening of the skull bones is accompanied by increasing size of the sinuses, and by a change in form of the sutures, from straight to wavy lines and finally to the complex interdigitations seen in the adult. After growth ceases, the skull sutures contain inert connective tissue and some cartilage; in old age some of them are completely replaced by bone (natural synostosis).
Bailey AP, Streit A. Sensory organs: making and breaking the pre-placodal region. Curr Top Dev Biol. 2006;72:167-204.
Barry A. The aortic arch derivatives in the human adult. Anat Rec. 1951;III:221-238.
Blechschmidt E. The Stages of Human Development Before Birth. Philadelphia: WB Saunders, 1961.
de la Cuadra-Blanco C, Peces-Peña MD, Jáñez-Escalada L, Mérida-Velasco JR. Morphogenesis of the human excretory lacrimal system. J Anat. 2006;209:127-135.
Ferguson MWF. The orofacial region. In: Wigglesworth JS, Singer DB. Textbook of Fetal and Perinatal Pathology. Oxford: Blackwell Scientific, 1991. Chapter 22.
Fukumoto S, Yamada Y. Extracellular matrix regulates tooth morphogenesis. Connect Tissue Res. 2005;46:220-226.
Gorlin RJ, Cohen MM, Hennekam RCM. Syndromes of the Head and Neck. 4th edn.. New York: Oxford University Press; 2001.
Hamilton WJ, Mossman HW. Human Embryology. Cambridge: Heffer, 1972.
Hinrichsen KV, editor. Human-Embryologie. Berlin: Springer-Verlag, 1990.
Jiang X, Iseki S, Maxson RE, Sucov HM, Morriss-Kay GM. Tissue origins and interactions in the mammalian skull vault. Dev Biol. 2002;241:106-116.
Jiang X, Rowitch DH, Soriano P, McMahon AP, Sucov HM. Fate of the mammalian cardiac neural crest. Development. 2000;127:1607-1616.
Matsuoka T, Ahlberg PE, Kessaris N, et al. Neural crest origins of the neck and shoulder. Nature. 2005;436:347-355.
Morriss-Kay GM, Wilkie AOMW. Growth of the normal skull vault and its alteration in craniosynostosis. J Anat. 2005;207:637-653.
O’Rahilly R, Müller F 1987 Developmental Stages in Human Embryos. Carnegie Institution of Washington Publication 637.
O’Rahilly R, Müller F. Human Embryology and Teratology. 3rd edn.. New York: Wiley-Liss; 2001.
O’Rahilly R, Müller F. The development of the neural crest in the human. J Anat. 2007;211:335-351.
Patten BM. Human Embryology. 3rd edn.. New York: Blakiston; 1968.
Santagati F, Rijli FM. Cranial neural crest and the building of the vertebrate head. Nat Rev Neurosci. 2003;4:806-820.
Thesleff I. Genetic basis of tooth development and dental defects. Acta Odontol Scand. 2000;58:191-194.
Wilkie AOMW, Morriss-Kay GM. Genetics of craniofacial development and malformation. Nat Rev Genet. 2001;2:458-468.