CHAPTER 41 Development of the eye
The development of the eye involves a series of inductive interactions between neighbouring tissues in the embryonic head. These are the neurectoderm of the forebrain (which forms the sensory retina and accessory pigmented structures), the surface ectoderm (which forms the lens and the corneal epithelium) and the intervening neural crest mesenchyme (which contributes to the fibrous coats of the eye and to tissues of the anterior segment of the eye). A broad anterior domain of neurectoderm characterized by the activation of several homeobox-containing transcriptional regulators, including PAX6, RX, SIX3 and OTX2, develops the potential to form optic vesicles. Subsequent interactions between mesenchyme and neurectoderm involving expression of the secreted protein SHH at the midline subdivides this eye field region into bilateral domains at the future sites of the eyes (Chow & Lang 2001). Loss of SHH function causes holoprosencephaly and a range of malformations that can include cyclopia. The parallel process of lens determination appears to depend on a brief period of inductive influence that spreads through the surface ectoderm from the rostral neural plate and elicits a lens-forming area of the head. Reciprocal interactions that are necessary for the complete development of both tissues take place as the optic vesicle forms and contacts the potential lens ectoderm (Saha et al 1992). Vascular tissue of the developing eye may form by local angiogenesis or vasculogenesis of angiogenetic mesenchyme. (Accounts of the development of the eye are given in O’Rahilly 1966 and 1983.)
EMBRYONIC COMPONENTS OF THE EYE
The first morphological sign of eye development is a thickening of the diencephalic neural folds at 29 days postovulation, when the embryo has seven to eight somites. This optic primordium (eye field) extends on both sides of the neural plate and crosses the midline at the primordium chiasmatis. A slight transverse indentation, the optic sulcus, appears in the inner surface of the optic primordium on each side of the brain. During the period when the rostral neuropore closes, at about 30 days (stage 11), the walls of diencephalon 1 (see Fig. 24.2) begin to evaginate at the optic sulcus, projecting laterally towards the surface ectoderm, so that, by 32 days, the optic vesicles are formed. Failure of the specification and development of the optic vesicle is associated with mutation of several transcriptional regulator genes expressed in the eye field and leads to anophthalmia (absence of the eye) (Graw 2003). The lumen of each optic vesicle is continuous with that of the neuromere diencephalon 1; the vesicle is surrounded by a sheath of mesenchymal cells derived from the head mesenchyme and neural crest. By 31 days, regional differentiation is apparent in each of the source tissues of the eye. The optic vesicle is visibly differentiated into its three primary parts: at the junction with the diencephalon, a thick-walled region marks the future optic stalk; laterally, the tissue which will become the sensory (neural) retina forms a flat disc of thickened epithelium in close contact with the surface ectoderm; the thin-walled part that lies between these regions will later form the pigmented layer of the retina (retinal pigmented epithelium). The area of surface ectoderm that is closely apposed to the distal optic vesicle thickens to form the lens placode, and the mesenchymal sheath of the vesicle begins to show signs of angiogenesis. Between 33 and 35 days postovulation, the lens placode and optic vesicle, responding to inductive signalling interactions involving fibroblast growth factors (FGFs) between these two tissues (Chow & Lang 2001), undergo coordinated morphogenesis. The lens placode invaginates, forming a pit which pinches off from the surface ectoderm to form the lens vesicle (Fig. 41.1). The surface ectoderm reforms a continuous layer which will become the corneal epithelium. The lateral part of the optic vesicle invaginates to form a cup: the inner layer (facing the lens vesicle) will become the sensory (neural) retina, and the outer layer, influenced by signals from the surrounding extra ocular mesenchyme, becomes the retinal pigmented epithelium. As a result of these folding movements, what were the apical (luminal) surfaces of the two layers of the cup now face one another across a much reduced lumen, the intraretinal space. The pigmented layer becomes attached to the mesenchymal sheath, but the junction between the pigmented and sensory layers is less firm and is the site of pathological detachment of the retina. The two layers are continuous at the lip of the cup (Fig. 41.2). The narrow part of the optic vesicle between the base of the cup and the brain forms the optic stalk. As well as the invagination of the lateral part of the optic vesicle, the ventral surface of the vesicle and distal part of the stalk similarly invaginate, forming a wide groove, the choroid (optic) fissure, through which mesenchyme and the hyaloid artery extend. These infoldings involve differential growth and cell movement, high and levels of proliferation in the inner neuroepithelial layer. As growth proceeds, the fissure closes and the artery is included in the distal part of the stalk. The fusion process is characterized by apoptosis at the margins of the fissure. Failure of the optic fissure to close is a rare anomaly that is accompanied by a corresponding deficiency in the choroid and iris (congenital coloboma) and is often associated with microphthalmia (small eyes). Reduced growth of the optic cup caused by mutation of the homeobox gene CHX10, which is important for specification and growth of the neural retina, is one known cause of microphthalmia (Graw 2003).
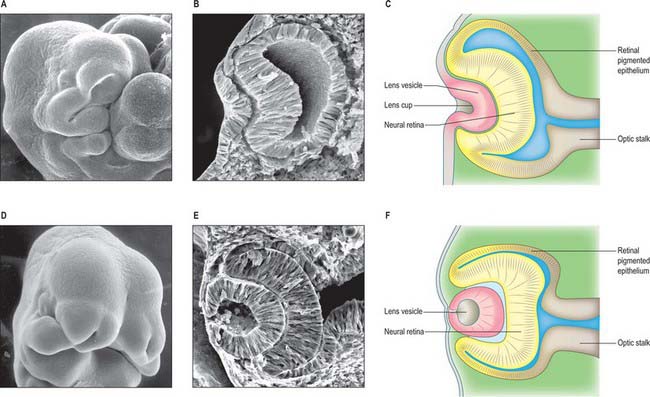
Fig. 41.1 Development and morphogenesis of the optic vesicle.
(A, B, D, E By courtesy of Kathleen Sulik PhD, Professor, University of North Carolina.)
DIFFERENTIATION OF THE FUNCTIONAL COMPONENTS OF THE EYE
The optic cup becomes patterned, from the base to the rim, into regions with distinct functions. Several secreted factors, including bone morphogenetic proteins (BMP), retinoic acid and SHH, and transcriptional regulators, including PAX6 and PAX2, are important for specifying each region (Chow & Lang 2001). The outer layer of the optic cup remains as a thin layer of cells which begin to acquire pigmented melanosomes and form the pigmented epithelium of the retina around 36 days. In a parallel process, which begins before invagination, the cells of the inner layer of the cup proliferate to form a thick pseudostratified neuroepithelium, the future neural retina, over the base and sides of the cup. The peripheral region around the lip of the cup is further differentiated into the components of the prospective iris at the rim, and the ciliary body a little further back adjacent to the neural retina (Fig. 41.2). The development of this pattern is reflected in regional differences in the expression of various genes which encode transcriptional regulators and which are therefore likely to play key roles in controlling and coordinating development. Distinct sets of genes are expressed prior to and during overt cell-type differentiation. For example, PAX6 is expressed in the prospective ciliary and iris regions of the optic cup: individuals heterozygous for mutations in PAX6 lack an iris (aniridia), which suggests a causal role for this gene in the development of the iris. The genes expressed in the eye are also often active at a variety of other specific sites in the embryo, which may, in part, account for the co-involvement of the eye and other organs in syndromes which result from single genetic lesions, e.g. PAX2 mutation causes coloboma and kidney defects, reflecting the sites of expression of the gene (Graw 2003).
Developing neural retina
The developing neural retina consists of an outer nuclear zone, which contains dividing neuroepithelial retinal progenitor cells, and an inner marginal zone, which is initially devoid of nuclei. At around 37 days, the cells of the nuclear zone invade the marginal zone, and by stage 18 (44 days) the nervous stratum of the retina consists of inner and outer neuroblastic layers. Cell lineage analyses have shown that seven retinal cell types are all derived from a common multipotential retinal progenitor cell. Different types of retinal cells are born (cease dividing) in a conserved sequence during development: ganglion cells, amacrine cells, cone photoreceptors and horizontal cells develop early, whereas bipolar cells, rod photoreceptors and Müller glial cells develop later (Cepko et al 1996). Newly born cells migrate from the apical (ventricular) surface to the appropriate cell layer in the developing retina, establishing its characteristic laminar structure. The developing ganglion cell layer first separates from the neuroblastic layers by formation of the inner plexiform layer. The inner nuclear layer containing developing amacrine, horizontal, bipolar and Müller glial cells then separates from the outer nuclear layer containing the developing rod and cone photoreceptors by formation of the outer plexiform layer. Mature retinal neurones first appear in the central part of the retina. By the eighth month all the named layers of the retina can be identified. However, the photoreceptor cells continue to differentiate after birth, generating an array of increasing resolution and sensitivity; the macula does not reach maturity until 15–45 months after birth (Hendrickson & Yuodelis 1984).
Lens
The lens develops from the lens vesicle (Figs 41.1F, 41.2A),. Initially this is a ball of actively proliferating epithelium, but by stage 16 there is a discernible difference between the thin anterior (i.e. outward-facing) epithelium and the thickened posterior epithelium. Cells of the posterior wall lengthen and fill the vesicle (Fig. 41.2B,C), reducing the original cavity to a slit by about 44 days. The posterior cells become filled with a very high concentration of proteins (crystallins) which render them transparent; they also become densely packed within the lens as primary lens fibres. Cells at the equatorial region of the lens elongate and contribute secondary lens fibres to the body of the lens in a process which continues into adult life, sustained by continued proliferation of cells in the anterior epithelium. The polarity and growth of the lens appear to depend on the differential distribution of soluble factors which promote either cell division or lens fibre differentiation and are present in the anterior chamber and vitreous humour respectively. Congenital cataracts can be associated with mutations in genes that encode structural lens proteins, particularly crystallin proteins, as well as genes that encode transcriptional regulatory factors expressed specifically in the lens, such as MAF and PITX3, that are needed for normal lens development and which also influence normal growth of the globe of the eye (Graw 2003).
Anterior segment
Mesenchymal cells of neural crest origin migrate anteriorly around the optic cup and between the surface ectoderm and the lens to contribute to the development of anterior segment structures, including the ciliary body, the iris, the cornea and the iridocorneal angle tissues (Fig. 41.2C–E) (Gould et al 2004). The anterior chamber initially appears as a cleft in this mesenchymal tissue. The mesenchyme superficial to the cleft forms the stroma (substantia propria) and endothelium of the cornea, and that deep to the cleft forms the stroma of the iris and the pupillary membrane. Tangentially, this early cleft extends as far as the iridocorneal angle, where communications are established with the scleral venous sinus (canal of Schlemm). Mesenchymal cells of neural crest origin lying at the angle of the anterior chamber differentiate to form a specialized meshwork of trabecular beams (collagen fibrils covered by cells): the open spaces of the meshwork become open to the anterior chamber as the beams develop. The canal of Schlemm develops deep to the trabecular meshwork and is derived from mesodermal mesenchyme. Initially a vascular structure lined by endothelial cells, the canal acts as an aqueous sinus from the fifth month of gestation. The forward-growing optic cup rim differentiates into the ciliary epithelium and the iris; the posterior chamber is formed between the iris, the lens capsule and the zonular suspensory fibres and the ciliary processes. The ciliary processes produce the aqueous humour that flows through the pupil and is drained in the iridocorneal angle mainly by the trabecular meshwork and the canal of Schlemm. In this way the walls of the anterior and posterior segment chambers furnish both the sites of production of, and the channels for circulation and reabsorption of, the aqueous humour. The FOXC1 and PITX2 transcriptional regulatory genes are expressed in the neural crest cells migrating into the presumptive anterior segment, and regulate differentiation of the anterior segment tissues. Anterior segment dysgenesis involving mal-formation of the iris, cornea and angle occurs when these genes are mutated (e.g. Axenfeld–Rieger syndrome) and is often associated with raised intraocular pressure and glaucoma (Gould et al 2004).
Cornea
The cornea is induced in front of the anterior chamber by the lens and optic cup. The corneal epithelium is formed from surface ectoderm, and the primordial corneal endothelium lining the front of the anterior chamber is formed from mesenchymal cells derived from the neural crest (Fig. 41.2D,E). Mesenchymal cells migrate between these layers and differentiate to form fibroblasts and keratocytes which secrete the extracellular matrix of the corneal stroma. A regular array of collagen fibres (lamellae) is established between these two layers and serves to reduce scattering of light entering the eye. The most anterior region of the stroma (Bowman’s layer) develops as an acellular zone packed with collagen fibrils which confer strength on the layer. From the third month, the endothelium is organized as a monolayer of cells and develops a strong laminated basal lamina (Descemet’s membrane) adjacent to the stroma. The endothelium maintains corneal trans-parency by regulating the water content of the stroma.
DIFFERENTIATION OF STRUCTURES AROUND THE EYE
Extraocular muscles
The extrinsic ocular muscles are derived from prechordal mesenchyme which ingresses at the primitive node very early in development. The prechordal cells lie at the rostral tip of the notochordal process (see Fig. 12.4); they remain mesenchymal after the notochordal process becomes epithelial and gains a basal lamina. The prechordal mesenchyme migrates laterally towards the paraxial mesenchyme. Although this is a singular origin for muscle, the early myogenic properties of these cells have been demonstrated experimentally; if transplanted into limb buds, the cells are able to develop into muscle tissue (Wachtler & Jacob 1986).
Early embryos develop bilateral premandibular, intermediate and caudal cavities in the head (previously described as preotic somites). The walls of the premandibular head cavities are lined by flat or cylindrical cells that do not exhibit the characteristics of a germinal epithelium. As the oculomotor nerve grows down to the level of the head cavity, a condensation of premuscle cells appears at its ventrolateral side and later subdivides into the blastemata of the muscles that the nerve will supply. Similar events occur in the intermediate head cavity (trochlear nerve and superior oblique) and the caudal head cavity (abducens nerve and lateral rectus) (see Figs 12.4 and 35.7).
Eyelids
The eyelids are formed as small cutaneous folds of surface ectoderm with a core of neural crest mesenchyme (Fig. 41.2C). During the middle of the third month, their edges come together and unite over the cornea to enclose the conjunctival sac; they usually remain united until about the end of the sixth month. When the eyelids open, the conjunctiva lining their inner surfaces and covering the white (scleral) region of the eye fuses with the corneal epithelium. Transforming growth factor alpha (TGF-α) and several other growth factors regulate the mesenchyme–epithelium interactions and cell migration that are required for eyelid formation; keratinization is thought to play an important role in lid separation. The eyelashes and lid glands, i.e. sebaceous glands associated with the eyelashes, and tarsal (Meibomian) glands develop from ectoderm, as do the lacrimal and accessory lacrimal glands. Orbicularis oculi, which closes the eyelids, develops on each side from skeletal myoblasts which invade the eyelids from the second pharyngeal arch. The muscles that widen the palpebral fissure develop within the orbit from mesenchymal cells: the superior and inferior tarsal muscles are smooth muscle, whereas levator palpebrae superioris is striated muscle that is attached to each upper eyelid by a tendon derived from the neural crest.
Lacrimal apparatus
The ectodermal epithelium of the superior conjunctival fornix proliferates and gives rise to a series of tubular buds which form the alveoli and ducts of the lacrimal gland. These buds are arranged in two groups: one forms the gland proper and the other forms its palpebral process (de la Cuadra-Blanco et al 2003). The lacrimal sac and nasolacrimal duct are derived from ectoderm in the nasomaxillary groove (between the lateral nasal process and the maxillary process of the developing face). The ectoderm thickens to form a solid cord of cells, the nasolacrimal ridge, which subsequently sinks into the mesenchyme and becomes canalized during the third month to form the nasolacrimal duct. The lacrimal canaliculi arise from the cranial extremity of the cord as buds which establish openings (puncta lacrimalia) on the margins of the lids. The inferior canaliculus isolates a small part of the lower eyelid to form the lacrimal caruncle and plica semilunaris.
Bharti K, Nguyen MT, Skuntz S, Bertuzzi S, Arnheiter H. The other pigment cell: specification and development of the pigmented epithelium of the vertebrate eye. Pigment Cell Res. 2006;19:390-394.
Cepko CL, Austin CP, Yang X, Alexiades M, Ezzeddine D. Cell fate determination in the vertebrate retina. Proc Natl Acad Sci USA. 1996;93:589-595.
Chow RL, Lang RA. Early eye development in vertebrates. Annu Rev Cell Dev Biol. 2001;17:255-296.
de la Cuadra-Blanco C, Peces-Peña MD, Mérida-Velasco JR. Morphogenesis of the human lacrimal gland. J Anat. 2003;203:531-536.
Gould DB, Smith RS, John SW. Anterior segment development relevant to glaucoma. Int J Dev Biol. 2004;48:1015-1029.
Graw J. The genetic and molecular basis of congenital eye defects. Nat Rev Genet. 2003;4:876-888.
Hendrickson AE, Yuodelis C. The morphological development of the human fovea. Ophthalmology. 1984;91:603-612.
O’Rahilly R. The early development of the eye in staged human embryos. Contrib Embryol Carnegie Inst. 1966;38:1.
O’Rahilly R. The timing and sequence of events in the development of the human eye and ear during the embryonic period proper. Anat Embryol. 1983;168:87-99.
Saha MS, Servetnick M, Grainger RM. Vertebrate eye development. Curr Opin Genet Dev. 1992;2:582-588.
Wachtler F, Jacob M. Origin and development of the cranial skeletal muscles. Bibl Anat. 1986;29:24-46.