CHAPTER 44 Development of the back
Vertebrae and their alternating intervertebral discs are one of the main manifestations of body segmentation or metamerism. A chain of segments arranged in sequence allows the overall structure to bend when it is moved by the associated muscles. The original body segments, the somites, are formed by the paraxial mesenchyme which is found lateral to the neural tube and notochord in the early embryo. The somites provide the embryonic cell populations for bone and muscle. The vertebrae form between the early body segments by the recombination of portions of the somites on the craniocaudal axis, and the muscles attach to adjacent vertebrae. Each vertebra develops from bilateral origins to form a midline centrum, two lateral arches bearing transverse processes which develop lateral and dorsal to the spinal cord, and a midline fused dorsal portion with a spinous process. Individual vertebrae may be distinguished by modifications of these component parts. The intervertebral discs develop from the same origins as the centra, and are composed of outer dense fibrous tissue surrounding a softer central zone.
SEGMENTATION OF PARAXIAL MESENCHYME
Epiblast cells which ingress through the lateral aspect of the primitive node and the rostral primitive streak (see Fig. 10.3) become committed to a somitic lineage (see p. 184). After passing through the streak the cells retain contact with both the epiblast and hypoblast basal laminae as they migrate and for some time after reaching their destination. The cells form populations of paraxial mesenchyme on each side of the notochord, termed presomitic or unsegmented mesenchyme. Somites will form from cultured presomitic mesenchyme with or without the presence of neural tube tissue or primitive node tissue. As well as specifying somitic lineage, the position of ingression of the epiblast informs the specific destination of the cells. Thus those cells which ingress through the lateral portion of Hensen’s node form the medial halves of the somites, whereas those ingressing through the primitive streak approximately 200 μm caudal to the node produce the lateral halves of the somites. The two somite halves do not appear to intermingle.
Experimental evidence (from chick embryos) shows that newly formed paraxial mesenchyme cells undergo 12 such cycles before they finally form a somite (Pourquie & Kusumi 2001). Thus from ingression through the primitive streak to segmentation into a somite takes approximately 18 hours. As the somite number varies between vertebrate species, it is likely that the rate of somite formation also varies and may be longer in human embryos. Indeed those vertebrates with elongated bodies and many somites appear to form somites more rapidly than those with shorter bodies, a finding which supports the concept that somite number is controlled in part by species-specific cyclical properties of the presomitic mesenchyme (Richardson et al 1998).
This area of research is moving rapidly. A detailed critique of the conceptual models of segmentation is given by Stern & Vasiliauskas (2000). An overview of the processes involved in the development of the paraxial mesenchyme, based on the work of Christ et al (2000), is shown in Figure 44.1.
SOMITOGENESIS
Somitogenesis is a process in which five main stages can be identified (Figs 44.2, 44.3). Compaction and formation of the spherical epithelial somite surrounding free somitocele cells occur prior to segmentation of the paraxial mesenchyme. Once the somite boundaries have been defined there is an epithelial/mesenchymal transition of the ventral and ventromedial walls of the somite to form the sclerotome. This is followed by bilateral movement of these ventral and medial mesenchyme populations around the notochord and the neural tube. The final stage is formation of the epithelial plate of the somite, also termed the dermomyotome, from the remaining somitic epithelium. Segmentation of the paraxial mesenchyme, formation of somites and resultant somitogenetic processes occur in a craniocaudal progression caudal to the otic vesicle from stage 9.
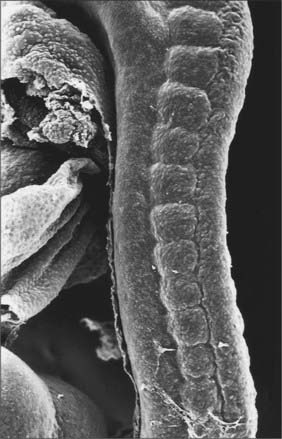
(Photograph by P Collins; printed by S Cox, Electron Microscopy Unit, Southampton General Hospital.)
The epithelial somite undergoes rapid development (Fig. 44.3). The cells of the ventromedial wall which were abutting the neural tube undergo an epithelial/mesenchyme transformation and break apart. The newly formed mesenchymal cells, which are collectively termed the sclerotome, proliferate. The remaining cells of the somite are now termed the epithelial plate of the somite (or dermomyotome). This epithelium is proliferative; it produces the cell lines which will give rise to (nearly) all the striated muscles of the body.
The sclerotomal population has been subdivided in the chick and the fates of the cells followed (Christ, Huang & Scaal 2004) (Fig. 44.4–44.6). The main mass of the sclerotome is termed the central sclerotome, that portion close to the notochord is termed the ventral sclerotome, and the portions adjacent to the dermomyotome are termed dorsal sclerotome and lateral sclerotome. The central sclerotome remains close to the dermomyotome; it will give rise to the pedicles and ventral parts of the neural arches, and the proximal ribs. The ventral sclerotomal cells, which were always lateral to the notochord, proliferate to form an axial cell population within the extracellular matrix of the perinotochordal space, now termed the perinotochordal sheath. The dorsal sclerotomal cells develop relatively late and invade the space between the surface ectoderm and growing neural tube and form the dorsal part of the neural arches. The lateral sclerotomal cells give rise to distal ribs and endothelial cells of blood vessels. Generally there is a dorsolateral expansion of the whole sclerotome rather than the medial migration of a population of sclerotomal cells (Gasser 2006). This can be seen in Figures 44.4–44.6. Sclerotomal cells also give rise to the meninges surrounding the spinal cord, local tendons and ligaments. The somitocele cells which remain mesenchymal throughout somite formation give rise to the vertebral joints, intervertebral discs and the proximal ribs (Christ, Huang & Scaal 2004).
The dermomyotome is a proliferative epithelium which produces cells from four borders (Fig. 44.7). Proliferation at the dorsomedial edge produces cells from the cranial to the caudal edge of the dermomyotome; they elongate beneath its basal lamina as they move laterally. Cells similarly proliferate from the cranial and caudal edges of the dermomyotome and these cells also elongate across it. The cells that are produced from these three edges are collectively termed the myotome, and will give rise to skeletal muscle dorsal to the vertebrae, i.e. the epaxial musculature. At limb levels cells de-epithelialize and migrate from the ventrolateral edges of the dermomyotome into the limb bud. Cells produced from this portion of the occipital somites migrate anteriorly to give rise to the intrinsic muscles of the tongue. At interlimb levels a hypaxial proliferation of myogenic cells that will give rise to intercostal and abdominal muscles extends from the ventrolateral edge of the epithelial dermomyotome into the body wall as development proceeds (Scaal & Christ 2004).
The regularity of somite formation provides criteria for staging embryos. The staging scheme proposed by Ordahl (1993) will be used in the following account of relative somite development. Ordahl noted that morphogenetic events occur in successive somites at approximately the same rate. The somite most recently formed from the unsegmented mesenchyme is designated as stage I, the next most recent as stage II, etc. After the embryo forms an additional somite, the ages of the previously formed somites increase by one Roman numeral. According to this scheme, compaction occurs at stage I; epithelialization at stages II to III; formation of mesenchymal sclerotome cells from stage V; myotome formation at stage VI; early migration of the ventrolateral lip of the epithelial plate and production of myotome cells are still occurring at stage X.
DEVELOPMENT OF SCLEROTOMES
Sclerotomal populations form from the ventral half of the epithelial somite. An intrasegmental boundary (fissure or cleft, sometimes termed von Ebner’s fissure) that is initially filled with extracellular matrix and a few cells, appears within the sclerotome and divides it into loosely packed cranial and densely packed caudal halves. The epithelial plate, and later the dermomyotome, spans the two half-sclerotomes. The bilateral sclerotomal cell populations migrate towards the notochord and surround it to form the perinotochordal sheath. They undergo a matrix-mediated interaction with the notochord, differentiating chondrogenetically to form the cartilaginous precursor of the vertebral centrum. The perinotochordal sheath transiently expresses type II collagen, and this is believed to initiate type II collagen expression, and thereafter a chondrogenic fate, in those mesenchyme cells which contact it. Each vertebra is formed by the combination of much of the caudal half of one bilateral pair of sclerotomes with much of the cranial half of the next caudal pair of sclerotomes. Their fusion around the notochord produces the blastemal centrum of the vertebra (Figs 44.8 and 44.9). The mesenchyme adjoining the intrasegmental sclerotomic fissure now increases greatly in density to form a well-defined perichordal disc which intervenes between the centra of two adjacent vertebrae and is the future anulus fibrosus of the intervertebral symphysis (‘disc’) (see below).
The basic pattern of a typical vertebra is initiated by this recombination of caudal and cranial sclerotome halves (Fig. 44.10), followed by differential growth and sculpturing of the sclerotomal mesenchyme which encases the notochord and neural tube. The centrum encloses the notochord and lies ventral to the neural tube. Condensation of the sclerotomal mesenchyme around the notochord and right and left neural processes can be seen in stage 15 human embryos. The neural processes curve to enclose the neural tube and extend to the dorsolateral angles of the centrum. The neural arch consists of paired bilateral pedicles (ventrolaterally) and laminae (dorsolaterally) which coalesce in the midline dorsal to the neural tube to form the spinous process. On each side three further processes project cranially, caudally and laterally from the junction of the pedicle and laminae. The cranial and caudal projections are the blastemal articular processes (zygapophyses) which become contiguous with reciprocal processes of adjacent vertebrae; their junctional zones mark the future zygapophysial or facet joints. The lateral projections are the true vertebral transverse processes. Bilateral costal processes (ribs) grow anterolaterally from the ventral part of the pedicles (i.e. near the centrum), from the neighbouring perichordal disc, and, at most thoracic levels, with accessions from the next adjacent caudal pedicles. The costal processes expand to meet the tips of the transverse processes. The definitive vertebral body is compound, and is formed from a median centrum (derived from the cells of the peri-notochordal sheath), and bilaterally from the expanded pedicle ends (derived from the migrating sclerotomal populations). These portions of the vertebral body fuse at the neurocentral synchondroses.
INTERVERTEBRAL DISCS
Vertebral centra are derived from caudal and cranial sclerotomal halves. An intervertebral disc is formed from the free somitocele cells within the epithelial somite which migrate with the caudal sclerotomal cells. The sclerotomal mesenchyme which forms the centra of the vertebrae replaces the notochordal tissue which it surrounds. In contrast, the notochord expands between the developing vertebrae as localized aggregates of cells and matrix which form the nucleus pulposus of the intervertebral disc (Figs 44.8 and 44.11). The intermediate part of each perichordal disc, which forms the anulus fibrosus, surrounds the nucleus pulposus and differentiates into an external laminated fibrous zone and an internal cuff (which lies next to the nucleus pulposus). The inner zone contributes to the growth of the outer. Near the end of the second month of embryonic life it begins to merge with the notochordal tissue, and is ultimately converted into fibrocartilage. After the sixth month of fetal life, notochordal cells in the nucleus pulposus degenerate, and are replaced by cells from the internal zone of the anulus fibrosus. This degeneration continues until the second decade of life, by which time all the notochordal cells have disappeared. In the adult, notochordal vestiges are limited, at the most, to non-cellular matrix.
(For further discussion of resegmentation theories, the reader is directed to Müller & O’Rahilly 1986; Huang et al 2000; Stern & Vasiliauskas 2000.)
DEVELOPMENT OF VERTEBRAE
The initial movements of sclerotomal cells round the neural tube and the expression of type II collagen signals the blastemal stage of vertebral development (Fig. 44.11). Chondrification begins at stage 17, initiating the cartilaginous stage. Each centrum chondrifies from one cartilage anlage. Each half of a neural arch is chondrified from a centre, starting in its base and extending dorsally into the laminae and ventrally into the pedicles, to meet, expand and blend with the centrum. By stage 23 there are 33 or 34 cartilaginous vertebrae, but the spinous processes have not yet developed, so the overall appearance is of total spina bifida occulta. Fusion of the spines does not occur until the fourth month. The transverse and articular processes are chondrified in continuity with the neural arches. Intervening zones of mesenchyme which do not become cartilage mark the sites of the facet joints and the complex of costovertebral joints, within which synovial cavities later appear.
A typical vertebra is ossified from three primary centres, one in each half vertebral arch and one in the centrum (see Fig. 42.24). Centres in arches appear at the roots of the transverse processes, and ossification spreads backwards into laminae and spines, forwards into pedicles and posterolateral parts of the body, laterally into transverse processes and upwards and downwards into articular processes. Classically centres in vertebral arches are said to appear first in upper cervical vertebrae in the ninth to 10th week, and then in successively lower vertebrae, reaching lower lumbar levels in the 12th week. However, in a radiographic study of unsexed human fetuses (Bagnall et al 1977), a pattern was noted which differed from such a simple craniocaudal sequence. A regular cervical progression was not observed. Centres first appeared in the lower cervical/upper thoracic region, quickly followed by others in the upper cervical region. After a short interval a third group appeared in the lower thoracolumbar region and remaining centres then appeared, spreading regularly and rapidly in craniocaudal directions.
During the first year the arches unite behind, first in the lumbar region and then throughout the thoracic and cervical regions. In upper typical cervical vertebrae, centra unite with arches about the third year, but in lower lumbar vertebrae, union is not complete until the sixth. The upper and lower surfaces of bodies and apices of transverse and spinous processes are cartilaginous until puberty, at which time five secondary centres appear, one in the apex of each transverse and spinous process and two annular epiphyses (‘ring apophyses’) for the circumferential parts of the upper and lower surfaces of the body. Costal articular facets are extensions of these anular epiphyses. They fuse with the rest of the bone at about 25 years. There are two secondary centres in bifid cervical spinous processes. Exceptions to this pattern of ossification are described in the appropriate subsections in Chapter 42.
Vertebrae are specified as to region very early in development. If a group of thoracic somites is transplanted to the cervical region, ribs will still develop. It is the sclerotome which is restricted: the myotome will produce muscle characteristic of the new location.
Occipitocervical junction
In humans, the junction between the head and neck (termed occipitocervical, craniovertebral or spinomedullary) is placed at the boundary between the fourth and fifth somites (Müller & O’Rahilly 1994). In avian embryos, where all embryonic stages may be obtained experimentally, the occipitocervical boundary has been determined as the fifth to sixth somite boundary (Wilting et al 1995). The boundary can first be determined in the human at stage 12 by the observation of hypoglossal nerve rootlets (Fig. 44.12). At stages 14 and 15 the junction is seen between the hypoglossal rootlets and the 1st spinal ganglion.
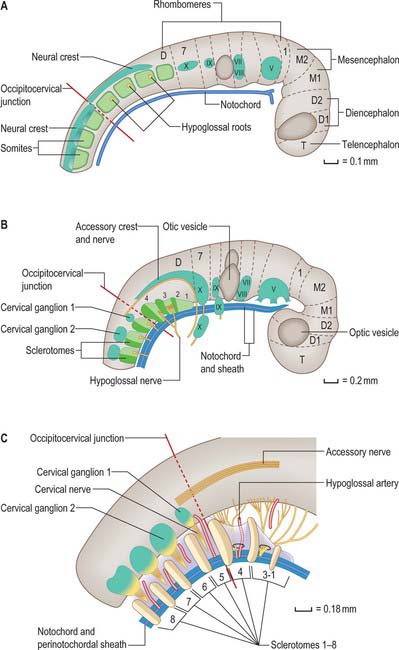
(After Müller F, O’Rahilly R 1994 Occipitocervical segmentation in staged human embryos. J Anat 185: 251–8. Permission by Blackwell Publishing.)
The first occipital somite disappears early and the caudal three fuse to form the basiocciput. Occipital sclerotomes 3 and 4 are the most distinct at stage 14, by which time the first three sclerotomes have fused. Vertebrae are formed from the fifth somite caudally: the first cervical vertebra is formed by the caudal half of occipital somite 4 and the cranial half of cervical somite 1 (Fig. 44.9). This shift of somite number and vertebral number accounts for the production of seven cervical vertebrae from eight cervical somites.
In the occipitocervical junctional region the centra formed from sclerotomes 5, 6 and 7 have a different fate from those more caudally placed. The lateral portions of these sclerotomes generally develop similarly to those of lower ones. In a study of occipitocervical segmentation in human embryos, Müller & O’Rahilly designated the three complete rostral centra which develop in the atlanto-axial region X, Y and Z (Fig. 44.13; Müller & O’Rahilly 1986). They noted that the height of the XYZ complex is equal to that of three centra elsewhere. X is on the level of sclerotome 5, and Y and Z are in line with sclerotome 6 and with the less dense portion of sclerotome 7. During stage 17 a temporary intervertebral disc appears peripherally between Y and Z. It begins to disappear in stage 21, although remains may be found in the adult. No disc develops between X and Y.
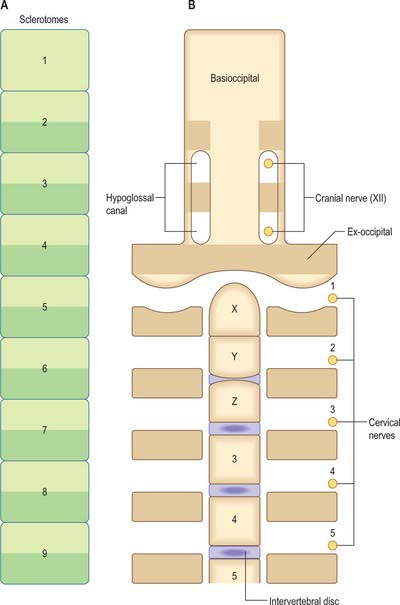
(After Müller F, O’Rahilly R 1994 Occipitocervical segmentation in staged human embryos. J Anat 185: 251–8. Permission by Blackwell Publishing.)
The origin of the anterior arch of the atlas is unclear. It is evident at stages 21–23 at the level of X or sometimes between X and Y. The posterior arch of the atlas arises from the dense area of sclerotome 5 at the level of X. The XYZ complex belongs to the axis, which means that the atlas does not incorporate a part of the central column (Müller & O’Rahilly 1994).
Decreased skull base height and diminished volume in the posterior fossa are structural anomalies associated with the Arnold–Chiari malformation. Although this is considered clinically to be a neurological defect, in that the medulla oblongata, and sometimes the cerebellar tonsils, project through the foramen magnum, there is good evidence that the underlying cause is a series of abnormalities of the occipitocervical junction. When the volume of the brain in the posterior cranial fossa and the volume of the fossa (delineated by bone and the tentorium cerebelli) were compared in controls and Arnold–Chiari cases, no significant difference was found between brain volume in the two groups, though the basiocciput, exocciput and supraocciput were significantly smaller in affected individuals (Nishikawa et al 1997). In patients with the Arnold–Chiari malformation, proatlas remnants and atlas assimilation are found. The sagittal canal diameter of the foramen magnum is critical: patients become symptomatic when this is less than 19 mm (Menezes 1995). Secondary skull base and cervical spine deformations, e.g. foramen magnum and cervical canal enlargement, may develop in association with the Arnold–Chiari malformation.
Abnormalities of the axis are usually concerned with fusion of the dens with the centrum of the second cervical sclerotomes. Using the classification of the three complete centra which develop in the atlanto-axial region as X, Y and Z (Müller & O’Rahilly 1986), failure of fusion of X with the YZ complex produces an ossiculum terminale, a dissociated apical odontoid epiphysis. Failure of fusion of the XY complex with Z at the dentocentral synchondrosis, or maintenance of the transitory intervertebral disc at this point, produces an os odontoideum, thought to be induced by excessive movement at the time of ossification of the dens (Crockard & Stevens 1995). Hypoplasia and aplasia of the X and Y centra, and aplasia of the Z centrum, will all lead to reduced size of the dens. There are widely differing views about whether this will lead to atlanto-axial instability.
C3–7, third to seventh cervical vertebrae
In cervical vertebrae 3–7 (Fig. 44.11) the transverse process is dorsomedial to the foramen transversarium. The costal process, corresponding to the head, neck and tubercle of a rib, limits the foramen ventrolaterally and dorsolaterally. The distal parts of these cervical costal processes do not normally develop; they do so occasionally in the case of the seventh cervical vertebra, and may even develop costovertebral joints. These cervical ribs may reach the sternum.
Thoracic vertebrae
Ribs
The costal processes attain their maximum length as the ribs in the thoracic region. Each rib originates from lateral sclerotomal populations, and forms from the caudal half of one sclerotome and the cranial half of the next subjacent sclerotome (Fig. 44.10). The head of the rib develops from somitocele cells from one somite, which migrate with the caudal half of the sclerotome. The proximal portion of the rib forms from both caudal and cranial sclerotomal halves; there is no mixing of cells from these origins. The distal portion of the rib forms from caudal and cranial sclerotomal halves; these cells mix as the rib extends into the ventral body wall and segmentation diminishes.
The ribs arise anterolaterally from the ventral part of the pedicles, and form bilateral costal processes which expand to meet the tips of the transverse processes. As they elongate laterally and ventrally they come to lie between the myotomic muscle plates. In the thorax (Fig. 44.11) the costal processes grow laterally to form a series of precartilaginous ribs. The transverse processes grow laterally behind the vertebral ends of the costal processes, at first connected by mesenchyme which later becomes differentiated into the ligaments and other tissues of the costotransverse joints. The capitular costovertebral joints are similarly formed from mesenchyme between the proximal end of the costal processes and the perichordal disc, and the adjacent parts of two (sometimes one) vertebral bodies, which are derived from the neural arch. Ribs 1–7 (vertebrosternal) curve round the body wall to reach the developing sternal plates. Ribs 8–10 (vertebrochondral) are progressively more oblique and shorter, only reaching the costal cartilage of the rib above, and contributing to the costal margin. Ribs 11 and 12 are free (floating), and have cone-shaped terminal cartilages to which muscles become attached (p. 921).
Lumbar vertebrae
The costal processes do not develop distally in lumbar vertebrae (Fig. 44.11). Their proximal parts become the ‘transverse processes’, while the morphologically true transverse processes may be represented by the accessory processes of the vertebrae. Occasionally, movable ribs may develop in association with the first lumbar vertebra.
Sacrum
Sacral vertebrae have lower centra and are narrower overall from side to side than their thoracic and lumbar counterparts. Each sacral vertebra is composed of a centrum and bilateral neural processes. The contribution of the costal processes to sacral development was examined by O’Rahilly et al (1990). These authors divided the neurocentral junctional area into two parts, anterolateral or alar, and posterolateral. They found the alar element in sacral vertebra 1 to be novel, since it was absent in lumbar vertebra 5. There is support for this view if the course of the dorsal rami of the spinal nerves is used to distinguish the costal elements ventrally from the transverse elements dorsally. The alar elements of the sacral vertebrae are ventral to the sacral dorsal rami, and both costal and transverse portions are posterolateral. The alar element of S1 and S2 forms the auricular surface of the sacrum. At stage 23 the cartilaginous sacral vertebrae have joined and the outline of the future bone can be recognized. Individual pedicles and laminae are very small and can be detected in S3–5.
Very rarely significant malformation of the sacral or lumbosacral vertebrae may develop, often in association with a maternal history of diabetes. When there is sacral agenesis, motor paralysis is profound below the affected vertebral level, whereas the sensory disturbance does not relate to the vertebral level so clearly and sensation may be present down to the knees. Bladder involvement is a consistent feature.
SPINA BIFIDA
Spina bifida is the generic term for a range of discrete defects of neurulation and subsequent vertebral formation. The spectrum of neural tube and vertebral defects includes a range of open neural tube defects: craniorachischisis (non-fusion of the entire neural tube and no vertebral arch development); anencephaly (non-fusion of the rostal portion of the neural tube with no calvarial or occipital development); and myelocele (non-fusion of caudal portions of the neural tube and local failure of vertebral arch development) (see Fig. 24.8).
DEVELOPMENT OF DERMOMYOTOMES
The dermomyotome, which forms from the dorsal half of the early somite, gives rise to all the skeletal muscle in the trunk and limbs and the intrinsic muscles of the tongue. Muscles in the head arise from the unsegmented paraxial mesenchyme rostral to the occipital somites. The dorsomedial lip (border) of the dermomyotome is an infolding of the epithelial plate of the somite and becomes a proliferative epithelial site from which mediolateral growth of the dermomyotome itself occurs. Three structures are required for myogenesis, the neural tube, the notochord/neural floor plate complex and the (early) dorsally placed ectoderm. There is a balance between levels of Shh from the notochord and Wnts from the dorsal neural tube and ectoderm which promotes myogenesis; high levels of either alone lead to sclerotomal or nonmyogenic dermomyotomal development (Scaal & Christ 2004). Myogenic determination factors, MyoD, myogenin, Myf 5 and herculin/MRF 4 can first be detected in the medial half of the somite as early as stage II, several hours prior to the onset of myotome formation.
The mode of formation of mononucleated, postmitotic, primitive myotubes from the dermomyotome has been elucidated in the chick but not yet confirmed in mammals (Gros, Scaal & Marcelle 2004). In this model all four borders of the dermomyotome give rise to myotomal cells which are produced in two phases: initially from the dorsomedial dermomyotomal lip by direct ingression and bidirectional extension; later, in a second phase, the caudal dermomyotomal border, followed in turn by the cranial dermomyotomal border and lastly the ventrolateral dermomyotomal lip, start to release myotomal precursor cells. Those myotomal cells arising from the dorsomedial dermomyotomal border contribute cells exclusively to the epaxial domain, those from the ventrolateral border contribute cells exclusively to the hypaxial domain, and the cells arising from the cranial and caudal borders move into both epaxial and hypaxial domains (Scaal & Christ 2004) (Fig. 44.7).
HYPAXIAL MUSCLE: VENTROLATERAL TRUNK MUSCLES
Two modes of production of hypaxial musculature are seen, related to axial level (Fig. 44.7). At cervical and trunk levels, myotubes are produced by the ventrolateral edge of the dermomyotome in a manner similar to the production of epaxial muscles. As development proceeds there is lateral growth of the dermomyotome so that the ventrolateral border extends ventrolaterally into the developing lateral and ventral body wall. The somite structures that invade the somatopleure of the abdominal and thoracic wall thus consist of two layers, dermomyotome and myotome, that give rise to the muscle blastemata for the intercostal and oblique muscles, transversus abdominis and rectus abdominis. At this time the number of somatopleural fibroblasts situated within the muscle-forming zone increases, and myotubes can be first seen. There is a subsequent ventral shift of the already separated muscle blastemata within the growing abdominal wall as they attain their definitive positions. Muscle differentiation continues and muscular connective tissue, tendons and aponeuroses develop.
At occipital and limb levels a ventrolateral dermomyotomal lip is not formed in the same way; cells undergo epithelial/mesenchymal transformation and migrate to their destination. Myoblasts move from the occipital dermomyotomes into the developing mandibular processes to form the intrinsic muscles of the tongue, whereas those opposite the early limb buds migrate into the limbs as dorsal and ventral muscle masses which give rise to the shoulder, hip and appendicular muscles. (For a review of dermomyotomal development see Scaal & Christ 2004.)
OTHER STRUCTURES DERIVED FROM THE SOMITES
Dermal precursors arise from the central portion of the dermomyotome and possibly also from the dorsomedial dermomyotome lip (Fig. 44.7). The cells undergo an epithelial/mesenchyme transformation from the dermomyotome and migrate to the dorsomedial subectodermal space overlying the dorsal neural tube. Their transformation seems to be controlled by factors from the neural tube. There is a sharp boundary between dermis which is somite-derived and that which is derived from the splachnopleuric mesenchyme of the lateral plate (and which covers the limbs, part of the lateral and all of the ventral body wall).
Smooth muscle cells within and around the developing somites and endothelial cell precursors are now considered to arise from somitic cells. All compartments of the epithelial somite, including the somitocele cells, give rise to angioblasts. The early ventral half of the somite gives rise to the endothelium of ventrolateral blood vessels. Angioblastic cells arising from the dorsomedial dermomyotome migrate mainly into the dorsal dermis, whereas those arising from the dorsolateral part move to the ventrolateral body wall and limbs (Scaal & Christ 2004).
Bagnall KM, Harris PF, Jones PRM. A radiographic study of the human spine II. The sequence of development of ossification centres in the vertebral column. J Anat. 1977;124:791-802.
Christ B, Huang R, Wilting J. The development of the avian vertebral column. Anat Embryol. 2000;202:179-194.
Christ B, Huang R, Scaal M. Formation and differentiation of the avian sclerotome. Anat Embryol. 2004;208:333-350.
Crockard HA, Stevens JM. Craniovertebral junction anomalies in inherited disorders: part of the syndrome or caused by the disorder? Eur J Pediatr. 1995;154:504-512.
Gasser RF. Evidence that some events of mammalian embryogenesis can result from differential growth, making migration unnecessary. Anat Rec. 2006;289B:53-63.
Gumpel-Pinot M. Muscle and skeleton of limbs and body wall. In: Le Douarin N, McLaren A. Chimeras in Developmental Biology. London: Academic Press; 1984:281-310.
Huang R, Zhi Q, Brand-Saberi B, Christ B. New experimental evidence for somite resegmentation. Anat Embryol. 2000;202:195-200.
Menezes AH. Primary craniovertebral anomalies and the hindbrain herniation syndrome (Chiari 1); database analysis. Pediatr Neurosurg. 1995;23:260-269.
Müller F, O’Rahilly R. Somitic-vertebral correlation and vertebral levels in the human embryo. Am J Anat. 1986;177:3-19.
Müller F, O’Rahilly R. Occipitocervical segmentation in staged human embryos. J Anat. 1994;185:251-258.
Nishikawa M, Sakamoto H, Hakuba A, Nakanishi N, Inoue Y. Pathogenesis of Chiari malformations: a morphometric study of the posterior cranial fossa. J Neurosurg. 1997;86:40-47.
O’Rahilly R, Müller F, Meyer DB. The human vertebral column at the end of the embryonic period proper. 4. The sacrococcygeal region. J Anat. 1990;168:95-111.
Ordahl CP. Myogenic lineages within the developing somite. In: Bernfield M, editor. Molecular Basis of Morphogenesis. New York: Wiley Liss; 1993:165-176.
Pourquie O, Kusumi K. When body segmentation goes wrong. Clin Genet. 2001;60:409-416.
Richardson MK, Allen SP, Wright GM, Raynaud A, Hanken J. Somite number and vertebrate evolution. Development. 1998;125:151-160.
Scaal M, Christ B. Formation and differentiation of the avian dermomyotome. Anat Embryol. 2004;208:411-424.
Stern CD, Vasiliauskas D. Segmentation: a view from the border. Current Topics in Developmental Biology. vol 47. New York: Academic Press; 2000.
Presents three different models of somite formation in the context of molecular data..
Wilting J, Ebensperger C, Müller TS, Kosecki H, Wallin J, Christ B. Pax-1 in the development of the cervico-occipital transitional zone. Anat Embryol. 1995;192:221-227.