CHAPTER 32 Coronary Calcium Assessment
IMPORTANCE OF CALCIUM IN IDENTIFYING CORONARY HEART DISEASE
In 2004, more than 15.8 million individuals in the United States developed CAD, and more than 450,000 died.1 Of the estimated 700,000 Americans who are expected to experience an acute coronary event this year, only 50% will have a prior history of CAD. About 17% of individuals who die of an acute coronary event are younger than 65 years. The estimated economic loss from coronary heart disease in 2007 was estimated to be greater than $151.6 billion, making CAD the largest single component of U.S. health care expenditures.
PATHOPHYSIOLOGY
Atherosclerotic Plaque Development
Coronary atherosclerotic plaque development begins early in life and is characterized by the accumulation of lipid-laden macrophages within the intima of arterial walls.2 With increasing accumulations, the lesions often progress to Stary type IV and type V atheroma, which are well-developed plaques characterized by intramural collections of cholesterol and phospholipids. These lipid collections are often covered by a thin, fibrous cap (fibroatheroma). Because of remodeling, the lesions initially have little significant luminal narrowing and are often undetected by angiography. Two thirds of individuals with acute myocardial infarctions or unstable angina have only minimal angiographic narrowing at the culprit site of occlusion. Myocardial perfusion studies that attempt to identify the hemodynamic effects of coronary stenoses may be normal and often underestimate an individual’s risk for a cardiac event.
A strong correlation has been found between the quantitative measurements of coronary artery calcium and pathologic measurements of plaque area and volume. Rumberger and colleagues3 showed that calcium is identifiable by CT when plaque area measures 5 to 10 mm2 per 3-mm-thick voxel. It has been established that as calcium increases, so does the likelihood of hemodynamically significant stenoses. Heavy concentrations of calcium suggest a greater atherosclerotic burden and a greater likelihood of hemodynamically significant stenoses. Supporting this concept is an article by Kragel and associates,4 who reported that atherosclerotic plaques associated with significant stenosis often contain more calcium than nonobstructive plaques.
Autopsy studies have shown that large CAC burdens correlate with greater likelihoods of significant arterial luminal narrowing, especially when distributed over multiple vessels. One such study was by Mautner and coworkers,5 who examined 1298 segments from 50 heart specimens and observed that 93% of arteries with stenoses greater than 75% had CAC. Conversely, only 14% of arteries with stenoses less than 25% were associated with calcification. Many other studies have shown that heavier CAC burdens were strongly associated with significant stenoses on angiography and with overall poorer patient outcomes. Calcium measurements derived from CT cannot predict site-specific stenoses. CAC measurements cannot be used to predict the site or the severity of the stenoses.6
Coronary calcium is a frequent constituent of vulnerable and hard plaques, and the presence and quantity of CAC correlate well with the overall severity of the atherosclerotic process, and make these lesions potentially identifiable by traditional noninvasive methods, such as fluoroscopy and CT. There are no diagnostic tests that can identify a priori vulnerable plaques that are susceptible to rupture. Postmortem analysis of coronary arteries of adults with sudden cardiac death have shown that histologically determined calcium scores for stable and ruptured plaques were similar (4.5 vs. 5.2).7 Despite this, plaque calcification is frequently present in most patients who have acute plaque disruption and sudden death. In addition, intravascular ultrasound examinations performed on patients with acute cardiac events (infarct or unstable angina) have shown that vulnerable plaques tend to be associated with less calcification than plaques found in patients with stable angina, and that moderate levels of coronary calcium portend a greater number of vulnerable plaques and a subsequent higher risk for sudden death. Complicating this issue further is evidence suggesting that small to moderate amounts of calcium within plaque may be associated with a more unstable plaque configuration, which may facilitate their eventual rupture, and may make plaques less tolerable to shear stresses and promote endothelial lining disruption.
IMAGING OF CORONARY ARTERY CALCIUM
CT imaging of small deposits of coronary calcium became available in the 1980s with the development of the electron-beam CT scanner. The electron-beam CT scanner has now been superseded by multidetector CT scanners, and few electron-beam CT scanners continue in operation. Multidetector CT scanners initially had a single detector ring technology, but now have progressed to dual source and 64-detector to 256-detector ring technology. Much of today’s coronary calcium imaging is being done on dual source and 64-detector CT scanners (Fig. 32-1).
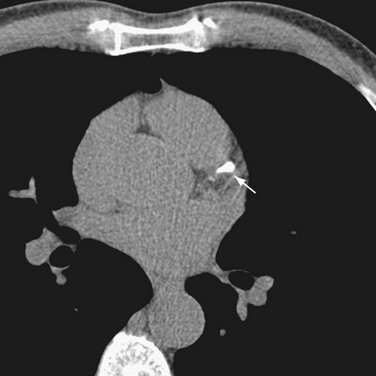
FIGURE 32-1 A 64-row multidetector CT image of a calcification (arrow) in the left anterior descending coronary artery.
Calcium Score Reporting
Calcium scores are reported using the Agatston score, volume score, and mass score.8 The Agatston score was the initial reporting score and is used in much of the older literature. It used electron-beam CT technology to identify lesions with a threshold of +130 Hounsfield units (HU) and 2 to 3 contiguous pixels located over the course of the coronary artery. To calculate the score, a region of interest is placed around each lesion, and the area of the lesion is multiplied by a weighted factor of 1 to 4 based on the peak signal anywhere in the lesion. A weighted factor of 1 is used for a peak calcification of 130 to 199 HU; 2, for a peak calcification of 200 to 299 HU; 3, for a peak calcification of 300 to 399 HU; and 4, for a peak calcification of greater than 400 HU.
The volume score linearly interpolates the data for isotropic volumes and represents the volume (in mm3) of each lesion above the 130 HU threshold. Lesions with similar area but differing amounts of calcium may have different volume scores.8
Rumberger and Kaufman8 reviewed the Agatston, volume, and mass scores, and found equivalence of the three CAC scoring methods for stratification of their cohort of 11,490 individuals who had undergone electron-beam CT. Likewise, comparable agreement was found among the three CAC scoring methods over successive electron-beam CT scans. Based on phantom experiments, however, these investigators reported nonlinearity of the Agatston and volume scores with the volume score overestimating lesion volume. Using the same phantoms, mass scores were found to be linear with a few exceptions.
Standardization of Computed Tomography Scanners
To ensure that calcium scores are meaningful, it is important that CT scanners and protocols are standardized so that scores from one scanner can be compared with another. Toward that end, the Physics Task Group of the International Consortium on Standardization in Cardiac CT was formed.9 Using a phantom with inserts of calcium and water density material embedded in an epoxy anthropomorphic body torso, scanning algorithms for all five commercially available scanners were developed (Fig. 32-2). The manufacturers included were Toshiba, Imatron, General Electric, Phillips, and Siemens.
RADIATION DOSAGE
For a multidetector CT prospectively triggered CAC study, the effective dose approximates 1.5 mSv in men and 1.8 mSv in women (6 months and 7.2 months of background radiation). If the multidetector CT CAC study were retrospectively gated, the radiation exposure would increase to approximately 3 mSv in men and 3.6 mSv in women, which would equate to 12 months and 14.4 months of background radiation. More recent developments in modulation techniques can now ramp down the power during noncritical imaging times, and this can decrease radiation exposure by 80%.10
Comparison of Multidetector Computed Tomography and Electron-Beam Computed Tomography in Coronary Artery Calcium Score Determinations
Numerous articles have compared multidetector CT and electron-beam CT in CAC score determinations. Knez and colleagues11 scanned 99 symptomatic men (mean age 60 years) with 4-detector multidetector CT and electron-beam CT (prospective triggering at 80% R–R interval) and found a correlation coefficient of r = 0.99 for volume and r = 0.98 for mass scoring. These investigators found a mean overall variability of 17%, but no significant differences for scores 1 to 100, 101 to 400, 401 to 1000, and greater than 1000. Knez and colleagues11 concluded that multidetector CT was equivalent to electron-beam CT for CAC scoring.
Becker and associates12 also compared 4-detector multidetector CT with electron-beam CT (prospective triggering) in 100 patients. They calculated Agatston, volume, and mass scores, and concluded that the variability was highest for the Agatston score (32%), and that the correlation between multidetector CT and electron-beam CT was excellent for volume and mass scores.
Horiguchi and colleagues13 compared electron-beam CT and 16-multidetector CT with retrospective gating in 100 patients and reported a high correlation between these scanners for the Agatston score (r2 = 0.95), volume score (r2 = 0.95), and mass score (r2 = 0.97). Last, Daniell and coworkers14 compared the results of electron-beam CT and 4-detector multidetector CT in 68 patients. Electron-beam CT and multidetector CT scores correlated well (r = 0.98-0.99). The variability between electron-beam CT and multidetector CT Agatston score was 25%, and for the volume score the variability was 16%. The electron-beam CT scores were higher than multidetector CT scores in approximately 50% of the cases. Numerous investigators have documented high correlation coefficients for multidetector CT versus electron-beam CT in coronary calcium scoring.
Interscan Reproducibility
A major consideration in the use of calcium scores is the variability of changes in CAC between serial CT scans on repeat imaging. Although interscan variability is predominantly affected by motion and table position, it is influenced further by the extent of CAC burden, scanner type, scan protocol starting position, and image artifacts. With optimal ECG triggering, variability can be reduced to less than 15% for electron-beam CT. The correlation coefficients of Agatston score, volume score, and mass score are excellent (r = 0.96).15 The conclusion was that the electron-beam CT and multidetector CT scanners have equivalent reproducibility.
Differences between scanner types have been reported in many studies. In the Multi-Ethnic Study of Atherosclerosis (MESA) trial of 6814 multiethnic individuals scanned on three electron-beam CT and three multidetector CT scanners, the differences were 20.1% for the Agatston score, and 18.3% for the interpolated volume score (P < .01).16 Other authors17 reported a multidetector CT variation of 12% for Agatston scores, 7.5% for volume scores, and 7.5% for mass scores. Overall, reported variabilities on 16-detector to 64-detector multidetector CT scanners seem to be in the range of 8% to 18% for the Agatston, volume, and mass scores.
CLINICAL IMPORTANCE OF CORONARY CALCIUM IMAGING
Coronary Artery Calcification as an Indicator of Coronary Stenosis
Many electron-beam CT studies have addressed the issue of CAC as an indicator of coronary stenosis. Haberl and coworkers18 looked at electron-beam CT calcium scores as an indicator of coronary luminal stenoses. They studied 1764 patients undergoing conventional coronary angiography and found that electron-beam CT CAC scores were a highly sensitive but only moderately specific determinant of stenosis. Knez and colleagues19 reviewed 16 electron-beam CT trials (N = 2115 patients) comparing electron-beam CT–derived Agatston scores with volume scores. The reference standard was conventional coronary angiography. Knez and colleagues19 found that electron-beam CT–derived CAC scores were an accurate predictor of the presence of greater than 50% luminal stenosis. Their results are shown in Table 32-1.
In another study, Budoff and colleagues20 analyzed electron-beam CT volume scores as a predictor of luminal stenoses in 1851 patients undergoing conventional coronary angiography. Their results are shown in Table 32-2. From these studies, the investigators concluded that electron-beam CT volume scores provided incremental value in predicting the severity and extent of angiographically significant coronary artery stenosis. Last, the American College of Cardiology/American Heart Association Expert Consensus Document on Electron-Beam CT for the Diagnosis and Prognosis of Coronary Artery Disease21 evaluated these and many additional studies, and reported a pooled sensitivity of 91.8% and specificity of 55% for detection of greater than 50% coronary artery stenoses.
TABLE 32-2 Results from Budoff et al: Presence of Significant Stenosis (>50% Stenosis)
Calcium Score | Sensitivity (%) | Specificity (%) |
---|---|---|
>20 | 90 | 58 |
>80 | 79 | 72 |
>100 | 76 | 75 |
Overall | 95 | 60 |
Value of Zero Calcium Score
More important than the total score may be the implications of a 0 calcium score. Several investigators have addressed this issue. Becker and associates22 studied 1347 symptomatic subjects with suspected CAD, and found an overall sensitivity of any calcium score to predict stenosis was 99%, with a specificity of 32%. An absolute score greater than or equal to 100 and an age-specific and gender-specific score greater than the 75th percentile were identified as the cutoff levels that provide the highest sensitivities (86% to 89%) and lowest false-positive rates (20% to 22%). Becker and associates22 also concluded that absence of coronary calcium was highly accurate for exclusion of CAD.
Several other studies have addressed this issue. Cheng and coworkers23 assessed the presence and severity of noncalcified coronary plaques on 64-detector multidetector CT versus coronary angiography in 554 symptomatic patients with low to intermediate pretest likelihood for CAD, and concluded that the absence of CAC is associated with a very low presence of significantly occlusive CAD.
Coronary Artery Calcium as a Predictor of Cardiac Events
In an American College of Cardiology/American Heart Association Consensus Document on Coronary Artery Calcium Scoring, it was concluded that CAC scores added incremental prognostic value in the evaluation of patients at intermediate risk for a coronary event. A study by Raggi and coworkers24 supported this conclusion. These investigators screened 632 patients with electron-beam CT for hard cardiac events (myocardial infarction and death), and found in 32-month follow-up that most events occurred in individuals with high calcium scores (>75th percentile) compared with age-matched and gender-matched controls. Arad and colleagues25 used electron-beam CT to screen 1172 asymptomatic patients. They followed patients for a mean of 3.6 years to determine the incidence of cardiovascular end points (myocardial infarction, death, and need for revascularization) and concluded that in asymptomatic adults electron-beam CT calcium scores were highly predictive of events.
Pletcher and associates26 reported a meta-analysis of studies between 1980 to 2003. In an analysis of 13,000 asymptomatic patients screened with electron-beam CT and followed for 3.6 years, they found the odds ratios for Agatston CAC scores less than 100, 100 to 400, and greater than 400 were 2.1, 4.2, and 7.2, and concluded that the electron-beam CT–derived Agatston calcium scores were independent predictors for hard coronary events in asymptomatic subjects. Several additional studies were reported in the American College of Cardiology Foundation/American Heart Association consensus document on CAC scoring, and all reported similar or better odds ratios for electron-beam CT as a predictor of a cardiac event.27
In patients experiencing acute hard cardiac events, coronary calcium is almost always present in amounts exceeding those found in asymptomatic individuals. Although acute cardiac events do occur in individuals who have little or no demonstrable calcium, the absence of detectable CAC does portend a low likelihood of a major cardiac event within the next 2 to 5 years (5% to 10% overall risk).21
IMPORTANCE OF CORONARY CALCIUM IN SPECIALIZED POPULATIONS
Risk Assessment in Asymptomatic Individuals
Present recommendations are that individuals at high risk are more likely to benefit from intensive risk modification and that individuals at low risk should adhere to a healthy lifestyle. In individuals at intermediate risk, the situation is uncertain, and further tests are often indicated. Calcium scoring has been advocated in the assessment of risk over measured conventional risk factors28,29 in intermediate-risk patients. Many authors believe it is reasonable to use CAC measurement as a treatment modifier in this group of patients.
Patients Presenting to Emergency Departments with Chest Pain
Laudon and colleagues30 performed CAC scoring in the emergency department in 104 patients, and reported a negative predictive value of 100% for a CAC score of 0. In another study, McLaughlin and associates31 reported a negative predictive value of 98% in 134 patients in a similar emergency department setting.31 Additionally, Georgiou and coworkers32 followed 198 patients presenting to the emergency department with chest pain and normal ECG and cardiac enzymes. They found that patients without CAC may safely be discharged from the emergency department because of the extremely low rate of future events (approximately 0.1%/yr). Numerous studies indicated the high negative predictive value of a 0 CAC score (r > 90%). The positive predictive value is lower, however, which results in CAC screening being a highly sensitive, but poorly specific modality for assessing patients with acute coronary syndrome.
More recently, Rubinshtein and coworkers33 assessed the severity of CAD using 64-multidetector CT in patients undergoing investigation for acute coronary syndrome. In 668 consecutive patients, 231 had a low (<100) or 0 CAC score, and of these patients, obstructive CAD was present in 9 of 125 (7%) with a 0 score, and in 18 of 106 (17%) with a low score (1 to 100). Rubinshtein and coworkers33 concluded a 0 CAC score seemed to be a good predictor for the exclusion of significant CAD in patients with intermediate to high pretest likelihood of obstructive CAD. Low CAC scores remain controversial, however, because many studies have shown that the presence of noncalcified and potentially obstructive lesions is higher in patients with low calcium scores compared with patients with a score of 0.
Relationship Between Race and Calcium Score
Many studies have shown differences in scores between men and women and between subjects of different ethnicities. Bild and colleagues,34 evaluating data from the MESA study, found that the prevalence of CAC (score >0) was highest in whites followed by Chinese, Hispanics, and blacks. Also from the MESA study of 6722 multiethnic individuals, Detrano and coworkers35 reported that CAC is a strong predictor of cardiovascular death, nonfatal myocardial infarction, angina, and need for revascularization. They concluded that CAC adds incremental prognostic value beyond traditional risk factors for the prediction of events. It seems appropriate to consider CAC as an indicator of increased risk in all races.
Elderly Populations
Currently, only a few studies have focused on the predictive value of CAC in elderly individuals. One of these was the Rotterdam Coronary Calcification Study, which evaluated older individuals with a mean age of 71 years. This study found that during a mean follow-up period of 3.3 years, 50 of the 1795 initially asymptomatic subjects had a coronary event.29 From these data, the researchers reported that increasing calcium scores showed relative risks for CAD up to 8.2 for a calcium score greater than 1000 compared with absent or low calcium score (0 to 100). Increasing calcium scores seem to be strongly associated with an increasing incidence of events, and low calcium scores seem to be important even in elderly individuals. Supporting these findings is a study by LaMonte and associates,36 in which CAD event rates were adjusted for gender. These investigators showed that in subjects older than 65 years there was a graded increase in event rates for calcium scores of 100 and 400 (7.1 and 8.2 per 1000 person-years). Conversely, the absence of coronary calcium was associated with a very low event rate (0.9 per 1000 person-years). There is support that CAC screening is valuable in all age groups.
Diabetes Mellitus and Coronary Artery Calcium Screening
It is well known that diabetes mellitus is associated with a high prevalence of CAD. Limited outcome data exist for diabetic patients, however. Anand and colleagues37 performed sequential CAC and myocardial perfusion imaging in 180 patients with type 2 diabetes. The incidence of myocardial ischemia was found to be proportional to the CAC score. For patients with type 2 diabetes with a CAC score of 0, 11 to 100, 101 to 400, 401 to 1000, and greater than 1000, the incidence of myocardial ischemia on stress perfusion imaging was 0%, 18%, 23%, 48%, and 71%. Anand and colleagues37 concluded that patients with a CAC score greater than 100 have an increased frequency of ischemia on myocardial perfusion imaging.
Raggi and coworkers38 evaluated 903 diabetic patients from a database of 10,377 asymptomatic individuals followed for an average of 5 years after CAC screening. The end point of the study was all-cause mortality. The authors showed that all-cause mortality was higher in diabetic patients than nondiabetic patients for any degree of CAC, and the risk increased as the score increased. They also found the absence of CAC predicted a low short-term risk of death (approximately 1% at 5 years) for diabetic patients and nondiabetic subjects. It seems that the presence and the absence of CAC are important modifiers of risk even in the presence of established risk factors.
Renal Failure and Coronary Artery Calcium
It is also well known that the prevalence of CAC increases with declining renal function. Russo and colleagues39 reported the prevalence of CAC to be 40% in 85 predialysis patients compared with 13% with normal renal function. In this prospective study of 313 high-risk hypertensive patients, a reduced glomerular filtration rate was shown to be the major determinant of the rate of progression of CAC. Consistent with these findings, Sigrist and associates40 reported prevalence of CAC to be 46% in 46 predialysis patients compared with 70% and 73% in 60 hemodialysis patients and 28 peritoneal dialysis patients (P = .02).
In another study of patients undergoing hemodialysis followed for almost 5 years, Block and colleagues41 reported a low mortality rate for patients with a negative calcium score (3.9%/yr). This is a remarkable finding in view of the extremely high mortality and cardiovascular event rate in these patients (approximately 25%/yr to 30%/yr). CAC seems to be predictive of an adverse outcome in renal dialysis patients; however, its absence is associated with a low event rate.
Comparison of Coronary Calcium Screening with Treadmill and Nuclear Stress Imaging
Exercise stress testing is frequently used in patients with suspected CAD. There are often numerous false-negative results, however. Lamont and colleagues42 studied 153 patients who underwent electron-beam CT and coronary angiography because of a positive treadmill stress test. In patients with a CAC score of 0, the negative predictive value was 93%. The authors concluded that the absence of CAC reliably identified patients with a false-positive treadmill stress test result. Raggi and coworkers43 also evaluated treadmill stress test and showed that in patients with low to intermediate pretest probabilities of disease, CAC scoring as the initial test provided a substantial cost benefit over exercise stress testing, and that a CAC score of 0 can reliably exclude CAD.
Berman and associates44 also reported a positive relationship between stress-induced myocardial ischemia as seen on single-photon emission computed tomography (SPECT) myocardial perfusion studies and electron-beam CT CAC. They studied 1195 patients without known coronary disease and found the frequency for ischemic SPECT was less than 2% with CAC scores less than 100 and increased progressively with CAC greater than 100 (P ≤ .0001). Symptomatic patients with CAC scores equal to or greater than 400 had a higher likelihood of myocardial ischemic changes versus asymptomatic patients (P < .025). Berman and associates44 concluded that ischemic SPECT was associated with a high likelihood of subclinical atherosclerosis, but is rarely seen in CAC scores less than 100, and that most low CAC scores would obviate the need for subsequent noninvasive testing.
CALCIUM SCORE PROGRESSION
Serial changes in CAC score have important implications for monitoring responses to therapy and in identifying patients with aggressive disease who are at higher risk for CAD. Progression of CAC is generally calculated as a percent or absolute change from the baseline score. Raggi and colleagues45 defined a change of greater than 15% as true progression. Annual CAC progression rates typically are 20% to 24% per year using either the Agatston or the volume score. Factors modifying the rate of change include the patient’s CAC score, gender, age, family history of premature CAD, ethnicity, diabetes, body mass index, hypertension, and renal insufficiency. Most patients exhibit a positive change in CAC scores over time. A few (29% to 34%) may exhibit no change, however, especially if they have a low Framingham Risk Score, are women, or are individuals with a 0 score.
EFFECT OF STATIN THERAPY ON CORONARY ARTERY CALCIUM PROGRESSION
Numerous studies have evaluated changes in CAC scores after treatment with statins. In observational studies, untreated patients had an average CAC progression of 36%, whereas patients receiving statin therapy had changes averaging 13%.46 Randomized trials have sometimes failed to confirm these findings, however, and comparisons of intensive versus moderate statin therapy have shown no differences in CAC progression. This lack of effect suggests that a longer observational period may be needed, and that statins may reduce cardiac events independent of any effect on calcified plaque. Despite this suggestion, several reports have noted that rapid increases in the CAC score are associated with worsening of clinical outcomes, including myocardial infarction.
Greenland P, Bonow RO, Brundage BH, et al. ACCF/AHA 2007 clinical expert consensus document on coronary artery calcium scoring by computed tomography in global cardiovascular risk assessment and in evaluation of patients with chest pain: a report of the American College of Cardiology Foundation Clinical Expert Consensus Task Force (ACCF/AHA Writing Committee to Update the 2000 Expert Consensus Document on Electron Beam Computed Tomography). Circulation. 2007;115:402-426.
1 American Heart Association. Heart Disease and Stroke Statistics: 2007 update. Dallas, TX: American Heart Association; 2007.
2 Stary HC, Chandler AB, Glagov S, et al. A definition of initial, fatty streak, and intermediate lesions of atherosclerosis. Circulation. 1994;89:2462-2478.
3 Rumberger JA, Simons DB, Fitzpatrick LA, et al. Coronary artery calcium area by electron-beam computed tomography and coronary atherosclerotic plaque area: a histopathologic correlative study. Circulation. 1995;92:2157-2162.
4 Kragel AH, Reddy SG, Wittes JT, et al. Morphometric analysis of the composition of the atherosclerotic plaques in the four major epicardial coronary arteries in acute myocardial infarction and in sudden coronary death. Circulation. 1989;80:1747-1756.
5 Mautner GC, Mautner SL, Froehlich J, et al. Coronary artery calcification: Assessment with electron beam CT and histomorphometric correlation. Radiology. 1994;192:619-623.
6 Bormann JL, Stanford W, Stenberg RG, et al. Ultrafast tomographic detection of coronary artery calcification as an indicator of stenosis. Am J Card Imaging. 1992;6:191-196.
7 Taylor AJ, Burke AP, O’Malley PG, et al. A comparison of the Framingham Risk Index, coronary artery calcification, and culprit plaque morphology in sudden cardiac death. Circulation. 2000;101:1243-1248.
8 Rumberger JA, Kaufman L. A Rosetta stone for coronary calcium risk stratification: Agatston, volume, and mass scores in 11,490 individuals. AJR Am J Roentgenol. 2003;181:743-748.
9 McCollough CH, Ulzheimer S, Halliburton SS, et al. Coronary artery calcium: a multi-institutional, multimanufacturer international standard for quantification at cardiac CT. Radiology. 2007;243:527-538.
10 Hunold P, Vogt FM, Schmermund A, et al. Radiation exposure during cardiac CT: effective doses at multi-detector row CT and electron-beam CT. Radiology. 2003;226:145-152.
11 Knez A, Becker C, Becker A, et al. Determination of coronary calcium with multi-slice spiral computed tomography: a comparative study with electron-beam CT. Int J Cardiovasc Imaging. 2002;18:295-303.
12 Becker CR, Kleffel T, Crispin A, et al. Coronary artery calcium measurement: agreement of multirow detector and electron beam CT. AJR Am J Roentgenol. 2001;176:1295-1298.
13 Horiguchi J, Yamamoto H, Akiyama Y, et al. Coronary artery calcium scoring using 16-MDCT and a retrospective ECG-gating reconstruction algorithm. AJR Am J Roentgenol. 2004;183:103-108.
14 Daniell AL, Wong ND, Friedman JD, et al. Concordance of coronary artery calcium estimates between MDCT and electron beam tomography. AJR Am J Roentgenol. 2005;185:1542-1545.
15 Hoffman U, Siebert U, Bull-Stewart A, et al. Evidence for lower variability of coronary artery calcium mineral mass measurements by multidetector computed tomography in a community-based cohort—consequences for progression studies. Eur J Radiol. 2006;57:396-402.
16 Detrano RC, Anderson M, Nelson J, et al. Coronary calcium measurements: effect of CT scanner type and calcium measure on rescan reproducibility-MESA study. Radiology. 2005;236:477-484.
17 Ohnesorge B, Flohr T, Fischback R, et al. Reproduction of coronary calcium quantification in repeat examinations with retrospective ECG-gated multisection spiral CT. Eur Radiol. 2002;12:1532-1540.
18 Haberl R, Becker A, Leber A, et al. Correlation of coronary calcification and angiographically documented stenoses in patients with suspected coronary artery disease: results of 1,764 patients. J Am Coll Cardiol. 2001;37:451-457.
19 Knez A, Becker A, Leber A, et al. Relation of coronary calcium scores by electron beam tomography to obstructive disease in 2,115 symptomatic patients. Am J Cardiol. 2004;93:1150-1152.
20 Budoff MJ, Diamond GA, Raggi P, et al. Continuous probabilistic prediction of angiographically significant coronary artery disease using electron beam tomography. Circulation. 2002;105:1791-1796.
21 O’Rourke RA, Brundage BH, Froelicher VF, et al. American College of Cardiology/American Heart Association Expert Consensus document on electron-beam computed tomography for the diagnosis and prognosis of coronary artery disease. Circulation. 2000;102:126-140.
22 Becker A, Leber A, White CW, et al. Multislice computed tomography for determination of coronary artery disease in a symptomatic patient population. Int J Cardiovasc Imaging. 2007;23:361-367.
23 Cheng VY, Lepor NE, Madyoon H, et al. Presence and severity of noncalcified coronary plaque on 64-slice computed tomographic coronary angiography in patients with zero and low coronary artery calcium. Am J Cardiol. 2007;99:1183-1186.
24 Raggi P, Callister TQ, Cooil B, et al. Identification of patients at increased risk of first unheralded acute myocardial infarction by electron-beam computed tomography. Circulation. 2000;101:850-855.
25 Arad Y, Spadaro LA, Goodman K, et al. Prediction of coronary events with electron beam computed tomography. J Am Coll Cardiol. 2000;36:1253-1260.
26 Pletcher MJ, Tice JA, Pignone M, et al. Using the coronary artery calcium score to predict coronary heart disease events: a systematic review and meta-analysis. Arch Intern Med. 2004;164:1285-1292.
27 Greenland P, Bonow RO, Brundage BH, et al. ACCF/AHA 2007 clinical expert consensus document on coronary artery calcium scoring by computed tomography in global cardiovascular risk assessment and in evaluation of patients with chest pain: a report of the American College of Cardiology Foundation Clinical Expert Consensus Task Force (ACCF/AHA Writing Committee to Update the 2000 Expert Consensus Document on Electron Beam Computed Tomography). Circulation. 2007;115:402-426.
28 Greenland P, LaBree L, Azen SP, et al. Coronary artery calcium score combined with Framingham score for risk prediction in asymptomatic individuals. JAMA. 2004;291:210-215.
29 Vliegenthart R, Oudkerk M, Hofman A, et al. Coronary calcification improves cardiovascular risk prediction in the elderly. Circulation. 2005;112:572-577.
30 Laudon DA, Vukov LF, Breen FJ, et al. Use of electron-beam computed tomography in the evaluation of chest pain patients in the emergency department. Ann Emerg Med. 1999;33:15-21.
31 McLaughlin VV, Balogh T, Rich S. Utility of electron beam computed tomography to stratify patients presenting to the emergency room with chest pain. Am J Cardiol. 1999;84:327-328. A328
32 Georgiou D, Budoff MJ, Kaufer E, et al. Screening patients with chest pain in the emergency department using electron beam tomography: a follow-up study. J Am Coll Cardiol. 2001;38:105-110.
33 Rubinshtein R, Gaspar T, Halon DA, et al. Prevalence and extent of obstructive coronary artery disease in patients with zero or low calcium score undergoing 64-slice cardiac multidetector computed tomography for evaluation of a chest pain syndrome. Am J Cardiol. 2007;99:472-475.
34 Bild DE, Detrano R, Peterson D, et al. Ethnic differences in coronary calcification: the Multi-Ethnic Study of Atherosclerosis (MESA). Circulation. 2005;111:1313-1320.
35 Detrano R, Guerci AD, Carr JJ, et al. Coronary calcium as a predictor of coronary events in four racial or ethnic groups. N Engl J Med. 2008;358:1336-1345.
36 LaMonte MJ, FitzGerald SJ, Church TS, et al. Coronary artery calcium score and coronary heart disease events in a large cohort of asymptomatic men and women. Am J Epidemiol. 2005;162:421-429.
37 Anand DV, Lim E, Hopkins D, et al. Risk stratification in uncomplicated type 2 diabetes: prospective evaluation of the combined use of coronary artery calcium imaging and selective myocardial perfusion scintigraphy. Eur Heart J. 2006;27:713-721.
38 Raggi P, Shaw LJ, Berman DS, et al. Prognostic value of coronary artery calcium screening in subjects with and without diabetes. J Am Coll Cardiol. 2004;43:1663-1669.
39 Russo D, Palmiero G, De Blasio AP, et al. Coronary artery calcification in patients with CRF not undergoing dialysis. Am J Kidney Dis. 2004;44:1024-1030.
40 Sigrist M, Bungay P, Taal MW, et al. Vascular calcification and cardiovascular function in chronic kidney disease. Nephrol Dial Transplant. 2006;21:707-714.
41 Block GA, Spiegel OM, Erlich J, et al. Effects of sevelamer and calcium on coronary artery calcification in patients new to hemodialysis. Clin Exp Nephrol. 2004;8:54-58.
42 Lamont DH, Budoff MJ, Shavelle R, et al. Coronary calcium scanning adds incremental value to patients with positive stress tests. Am Heart J. 2002;143:861-867.
43 Raggi P, Callister TQ, Cooil B, et al. Evaluation of chest pain in patients with low to intermediate pretest probability of coronary artery disease by electron beam computed tomography. Am J Cardiol. 2000;85:283-288.
44 Berman DS, Wong ND, Gransar H, et al. Relationship between stress-induced myocardial ischemia and atherosclerosis measured by coronary calcium tomography. J Am Coll Cardiol. 2004;44:923-930.
45 Raggi P, Cooil B, Ratti C, et al. Progression of coronary artery calcium and occurrence of myocardial infarction in patients with and without diabetes mellitus. Hypertension. 2005;46:238-243.
46 Callister TQ, Raggi P, Cooil B, et al. Effect of HMG-CoA reductase inhibitors on coronary artery disease as assessed by electron-beam computed tomography. N Engl J Med. 1998;339:1972-1978.