Chapter 16 Cerebral Hemispheres
The cerebral hemispheres are the largest part of the human brain. They each have a highly convoluted external cortex, beneath which lies an extensive internal mass of white matter that contains the basal ganglia. Each hemisphere also contains a lateral ventricle, continuous with the third ventricle through the interventricular foramen. The two hemispheres are linked by the commissural fibres of the corpus callosum.
Gyri, Sulci, and Lobes
On the superolateral cerebral surface, two prominent furrows—the lateral (Sylvian) fissure and the central sulcus—are the main features that determine its surface divisions (Figs 16.1, 16.3). The lateral fissure is a deep cleft on the lateral and inferior surfaces. It separates the frontal and parietal lobes above from the temporal lobe below. It has a short stem that divides into three rami. The stem commences inferiorly at the anterior perforated substance, extending laterally between the orbital surface of the frontal lobe and the anterior pole of the temporal lobe and accommodating the sphenoparietal venous sinus. Upon reaching the lateral surface of the hemisphere, it divides into anterior horizontal, anterior ascending and posterior rami. The anterior ramus runs forward for 2.5 cm or less into the inferior frontal gyrus, and the ascending ramus ascends for an equal distance into the same gyrus. The posterior ramus is the largest. It runs posteriorly and slightly upward, across the lateral surface of the hemisphere for approximately 7 cm, and turns up to end in the parietal lobe. Its floor is the insula, and it accommodates the middle cerebral vessels.
The central sulcus (see Figs 16.1, 16.3) is the boundary between the frontal and parietal lobes. It starts in or near the superomedial border of the hemisphere, a little behind the midpoint between the frontal and occipital poles. It runs sinuously downward and forward for 8 to 10 cm to end a little above the posterior ramus of the lateral sulcus, from which it is always separated by an arched gyrus. Its general direction makes an angle of approximately 70° with the median plane. It demarcates the primary motor and somatosensory areas of the cortex, located in the precentral and postcentral gyri, respectively.
The medial cerebral surface (Figs 16.2, 16.4) lies within the great longitudinal fissure. The commissural fibres of the corpus callosum lie in the depths of the fissure. The curved anterior part of the corpus callosum is the genu, continuous below with the rostrum and narrowing rapidly as it passes back to the upper end of the lamina terminalis. The genu continues above into the trunk or body, the main part of the commissure, which arches up and back to a thick, rounded posterior extremity, the splenium. The bilateral vertical laminae of the septum pellucidum are attached to the concave surfaces of the trunk, genu and rostrum, occupying the interval between them and the fornix. In front of the lamina terminalis, and almost coextensive with it, is the paraterminal gyrus, a narrow triangle of grey matter separated from the rest of the cortex by a shallow posterior paraolfactory sulcus. A short vertical sulcus, the anterior paraolfactory sulcus, may occur a little anterior to the paraterminal gyrus. The cortex between these two sulci is the subcallosal area (paraolfactory gyrus).
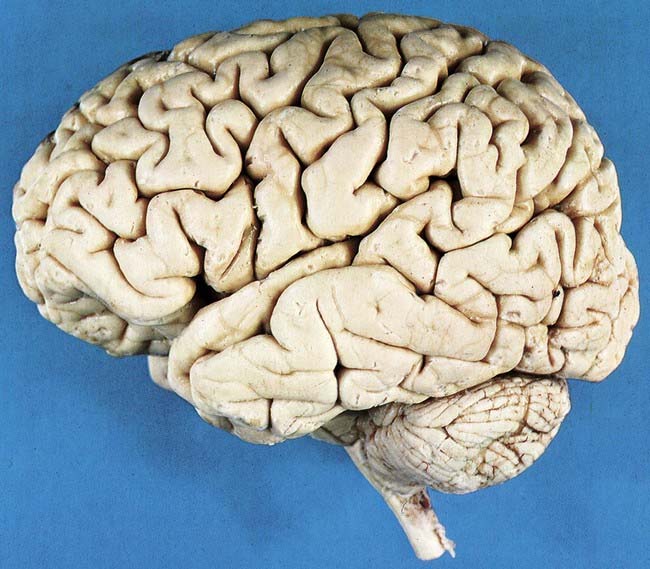
Fig. 16.3 Left lateral aspect of the brain.
(Dissection by E. L. Rees; photograph by Kevin Fitzpatrick on behalf of GKT School of Medicine, London.)
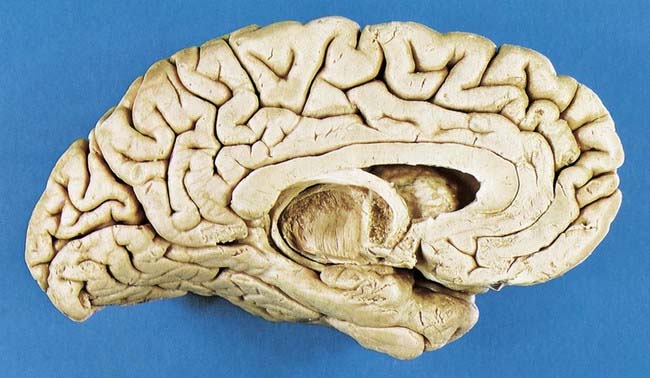
Fig. 16.4 Medial surface of the left cerebral hemisphere after sagittal section of the brain, followed by removal of the brain stem and septum pellucidum.
(Photograph by Kevin Fitzpatrick on behalf of GKT School of Medicine, London.)
The posterior region of the medial surface is traversed by the parieto-occipital and calcarine sulci. These two deep sulci converge anteriorly to meet a little posterior to the splenium. The parieto-occipital sulcus marks the boundary between the parietal and occipital lobes. It starts on the superomedial margin of the hemisphere approximately 5 cm anterior to the occipital pole, sloping down and slightly forward to the calcarine sulcus. The calcarine sulcus starts near the occipital pole. Although usually restricted to the medial surface, its posterior end may reach the lateral surface. Directed anteriorly, it joins the parieto-occipital sulcus at an acute angle behind the splenium. Continuing forward, it crosses the inferomedial margin of the hemisphere and forms the inferolateral boundary of the isthmus, which connects the cingulate with the parahippocampal gyrus. The visual cortex lies above and below the posterior part of the calcarine sulcus, behind the junction with the parieto-occipital sulcus. The calcarine is deep and produces an elevation, the calcar avis, in the wall of the posterior horn of the lateral ventricle.
The inferior cerebral surface is divided by the stem of the lateral fissure into a small anterior part and a larger posterior part (Figs 1.8, 16.5, 16.6). The anterior part is the orbital region of the inferior surface. It is transversely concave and lies above the cribriform plate of the ethmoid, the orbital plate of the frontal and the lesser wing of the sphenoid. A rostrocaudal olfactory sulcus traverses the region near its medial margin, overlapped by the olfactory bulb and tract. The medial strip thus demarcated is the gyrus rectus. The rest of this surface bears irregular orbital sulci, generally H-shaped, that divide it into the anterior, medial, posterior and lateral orbital gyri.
The larger posterior region of the inferior cerebral surface is partly superior to the tentorium as well as to the middle cranial fossa and is traversed by the anteroposterior collateral and occipitotemporal sulci (see Figs 16.2, 16.4). The collateral sulcus starts near the occipital pole and extends anteriorly and parallel to the calcarine sulcus, separated from it by the lingual gyrus. Anteriorly, it may continue into the rhinal sulcus, but the two are usually separate. The rhinal sulcus (fissure) runs forward in the line of the collateral sulcus, separating the temporal pole from the hook-shaped uncus posteromedial to it. This sulcus is the lateral limit of the piriform lobe (Fig. 16.7).
Cerebral Cortex
Microstructure
The neocortex essentially consists of three neuronal cell types. The most abundant are pyramidal cells. Non-pyramidal cells, also called stellate or granule cells, are divided into spiny and non-spiny neurones. All types have been subdivided on the basis of size and shape (Fig. 16.8).
Pyramidal cells (Fig. 16.9) have a flask-shaped or triangular cell body ranging from 10 to 80 µm in diameter. The soma gives rise to a single thick apical dendrite and multiple basal dendrites. The apical dendrite ascends toward the cortical surface, tapering and branching, to end in a spray of terminal twigs in the most superficial lamina, the molecular layer. From the basal surface of the cell body, dendrites spread more horizontally, for distances up to 1 mm for the largest pyramidal cells. Like the apical dendrite, the basal dendrites branch profusely along their length. All pyramidal cell dendrites are studded with myriad dendritic spines. These become more numerous as the distance from the parent cell soma increases. A single slender axon arises from the axon hillock, which is usually situated centrally on the basal surface of the pyramidal neurone. Ultimately, in the vast majority of (if not all) cases, the axon leaves the cortical grey matter to enter the white matter. Pyramidal cells are thus, perhaps universally, projection neurones. They appear to use excitatory amino acids, either glutamate or aspartate, exclusively as their neurotransmitters.
Spiny stellate cells are the second most numerous cell type in the neocortex and, for the most part, occupy lamina IV. They have relatively small multipolar cell bodies, commonly 6 to 10 µm in diameter. Several primary dendrites, profusely covered in spines, radiate for varying distances from the cell body. Their axons ramify within the grey matter, predominantly in the vertical plane. Spiny stellate cells are likely to use glutamate as their neurotransmitter.
Neurones with mainly horizontally dispersed axons include basket and horizontal cells. Basket cells have a short, vertical axon that rapidly divides into horizontal collaterals; these end in large terminal sprays synapsing with the somata and proximal dendrites of pyramidal cells. The cell bodies of horizontal cells lie mainly at the superficial border of lamina II and occasionally deep in lamina I (the molecular or plexiform layer). They are small and fusiform, and their dendrites spread short distances in two opposite directions in lamina I. Their axons often stem from a dendrite, then divide into two branches that travel away from each other for great distances in the same layer.
Laminar Organization
Typical neocortex is described as having six layers or laminae lying parallel to the surface (Figs 16.10–16.12).
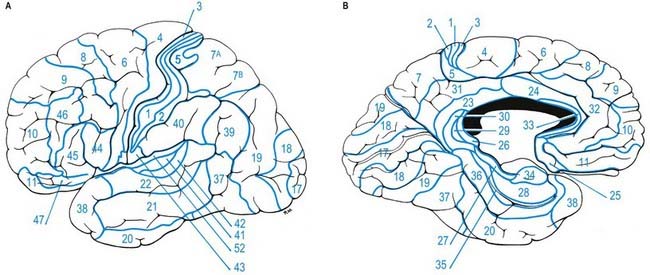
Fig. 16.12 Lateral (A) and medial (B) surfaces of the left cerebral hemisphere depicting Brodmann’s areas.
Neocortical Structure
Five regional variations are described in neocortical structure (see Fig. 16.11). Although all are said to develop from the same six-layered pattern, two types—granular and agranular—are regarded as virtually lacking certain laminae and are referred to as heterotypical. Homotypical variants, in which all six laminae are found, are called frontal, parietal and polar—names that link them with specific cortical regions in a somewhat misleading manner (e.g. the frontal type also occurs in parietal and temporal lobes).
The other three types of cortex are intermediate forms. In the frontal type, large numbers of small and medium-sized pyramidal neurones appear in laminae III and V, and the granular layers (II and IV) are less prominent. The relative prominence of these major forms of neurone vary reciprocally wherever this form of cortex exists.
For almost 100 years it has been customary to refer to discrete cortical territories not only by their anatomical location in relation to gyri and sulci but also in relation to their cytoarchitectonic characteristics as originally descried by Brodmann (see Fig. 16.12). Some of the areas so defined (e.g. the primary sensory and motor cortices) have clear relevance in terms of anatomical connections and functional significance; others less so.
Overview of Cortical Connectivity
All neocortical areas are connected with subcortical regions, although the density of these connections varies among areas. First among these are connections with the thalamus (Ch. 15). All areas of the neocortex receive afferents from more than one thalamic nucleus, and all such connections are reciprocal. The vast majority of (if not all) cortical areas project to the striatum, tectum, pons and brain stem reticular formation. Additionally, all cortical areas are reciprocally connected with the claustrum; the frontal cortex connects with the anterior part, and the occipital lobe with the posterior part.
Widely separated but functionally interconnected areas of cortex share common patterns of connections with subcortical nuclei and within the neocortex. For example, contiguous zones of the striatum, thalamus, claustrum, cholinergic basal forebrain, superior colliculus and pontine nuclei connect with anatomically widely separated areas in the prefrontal and parietal cortices, which are themselves interconnected. In contrast, other cortical regions that are functionally distinct (e.g. areas in the temporal and parietal cortices) do not share such contiguity in their subcortical connections. (See Case 12.)
Frontal Lobe
The frontal lobe is the rostral region of the hemisphere, anterior to the central sulcus and above the lateral fissure. On the superolateral surface, extending onto the medial surface, is the precentral gyrus, running parallel to the central sulcus and limited anteriorly by the precentral sulcus. The area of the frontal lobe anterior to the precentral sulcus is divided into the superior, middle and inferior frontal gyri (see Figs 16.1, 16.2). In front of these gyri lies the frontal pole. The ventral surface of the frontal lobe overlies the bony orbit and is the orbitofrontal cortex. The medial surface extends from the frontal pole anteriorly to the paracentral lobule behind. It consists of the medial frontal cortex and the anterior cingulate cortex.
Primary Motor Cortex
The primary motor cortex (MI) corresponds to the precentral gyrus (area 4). It is the area of cortex with the lowest threshold for eliciting contralateral muscle contraction by electrical stimulation. The primary motor cortex contains a detailed, topographically organized map (motor homunculus) of the opposite body half, with the head represented most laterally and the legs and feet represented on the medial surface of the hemisphere in the paracentral lobule (Fig. 16.13). A striking feature is the disproportionate representation of body parts in relation to their physical size. Thus, large areas represent the muscles of the hand and face, which are capable of finely controlled or fractionated movements.
The ipsilateral somatosensory cortex (SI) projects in a topographically organized way to area 4, and the connection is reciprocal. The projection to the motor cortex arises in areas 1 and 2, with little or no contribution from area 3b. Fibres from SI terminate in layers II and III of area 4, where they contact mainly pyramidal neurones. Evidence suggests that neurones activated monosynaptically by fibres from SI, as well as those activated polysynaptically, make contact with layer V pyramidal cells, which give rise to corticospinal fibres, including Betz cells. Movement-related neurones in the motor cortex that can be activated from SI tend to have a late onset of activity, mainly during the execution of movement. It has been suggested that this pathway plays a role primarily in the making of motor adjustments during a movement. Additional ipsilateral corticocortical fibres to area 4 from behind the central sulcus come from the second somatosensory area (SII).
Premotor Cortex
Immediately in front of the primary motor cortex lies Brodmann’s area 6 (Fig. 16.14). Area 6 extends onto the medial surface, where it becomes contiguous with area 24 in the cingulate gyrus, anterior and inferior to the paracentral lobule. A number of functional motor areas are contained in this cortical region. Lateral area 6, the area over most of the lateral surface of the hemisphere, corresponds to the premotor cortex. The premotor cortex is divided into dorsal and ventral areas on functional grounds and on the basis of ipsilateral corticocortical association connections.
Frontal Eye Field
The frontal eye field lies predominantly within Brodmann’s area 8, anterior to the superior premotor cortex (Fig. 16.15). It receives its major thalamic projection from the parvocellular mediodorsal nucleus, with additional afferents from the medial pulvinar, the ventral anterior nucleus and the suprageniculate–limitans complex. It connects with the paracentral nucleus of the intralaminar group. The thalamocortical pathways to the frontal eye field form part of a pathway from the superior colliculus, the substantia nigra and the dentate nucleus of the cerebellum. The frontal eye field has extensive ipsilateral corticocortical connections, receiving fibres from several visual areas in the occipital, parietal and temporal lobes, including the medial temporal area (V5) and area 7a. There is also a projection from the superior temporal gyrus, which is auditory rather than visual in function. From within the frontal lobe, the frontal eye field receives fibres from the ventrolateral and dorsolateral prefrontal cortices. It projects to the dorsal and ventral premotor cortices and to the medial motor area, probably to the supplementary eye field adjacent to the supplementary motor area proper. It projects prominently to the superior colliculus, the pontine gaze centre within the pontine reticular formation and other oculomotor-related nuclei in the brain stem. As its name implies, it is important in the control of eye movements. Destructive lesions of the frontal eye field cause ipsilateral conjugate deviation of the eyes, whereas stimulation, such as with an epileptic discharge, induces contralateral deviation.
Supplementary Motor Cortex
The supplementary motor area contains a representation of the body in which the leg is posterior and the face anterior, with the upper limb between them. Its role in the control of movement involves primarily complex tasks, which require temporal organization of sequential movements and retrieval of motor memory. The consequences of damage to the supplementary motor area bear some striking similarities to the effects of basal ganglia dysfunction; akinesia is common, and there may be problems with the performance of sequential, complex movements. Stimulation of the supplementary motor area in conscious patients has been reported to elicit the sensation of an urge to move or the feeling that a movement is about to occur. A region anterior to the supplementary motor area for face representation is important in vocalization and speech production.
Prefrontal Cortex
The prefrontal cortex on the lateral surface of the hemisphere comprises predominantly Brodmann’s areas 9, 46 and 45 (see Figs 16.12, 16.14, 16.15). In non-human primates, two subdivisions of the lateral prefrontal cortex are recognized: a dorsal area equivalent to area 9 and perhaps including the superior part of area 46, and a ventral area consisting of the inferior part of area 46 and area 45. Areas 44 and 45 are particularly notable in humans because, in the dominant hemisphere, they constitute the motor speech area (Broca’s area; see Case 12). Both the dorsolateral and ventrolateral prefrontal areas receive their major thalamic afferents from the mediodorsal nucleus, and there are additional contributions from the medial pulvinar, the ventral anterior nucleus and the paracentral nucleus of the anterior intralaminar group. The dorsolateral area receives long association fibres from the posterior and middle superior temporal gyrus, including auditory association areas; from parietal area 7a; and from much of the middle temporal cortex. From within the frontal lobe it also receives projections from the frontal pole (area 10) and from the medial prefrontal cortex (area 32) on the medial surface of the hemisphere. It projects to the supplementary motor area, the dorsal premotor cortex and the frontal eye field. All these thalamic and corticocortical connections are reciprocal. Commissural connections are with the homologous area and with the contralateral inferior parietal cortex. The ventrolateral prefrontal area receives long association fibres from areas 7a and 7b of the parietal lobe, auditory association areas of the temporal operculum, the insula and the anterior part of the lower bank of the superior temporal sulcus. From within the frontal lobe it receives fibres from the anterior orbitofrontal cortex and projects to the frontal eye field and the ventral premotor cortex. It connects with the contralateral homologous area via the corpus callosum. These connections are probably all reciprocal.
Parietal Lobe
The lateral aspect of the parietal lobe is divided into three areas by postcentral and intraparietal sulci (see Fig. 16.1). The postcentral sulcus, often divided into upper and lower parts, is posterior and parallel to the central sulcus. Inferiorly, it ends above the posterior ramus of the lateral fissure. The postcentral gyrus or primary somatosensory cortex lies between the central and postcentral sulci. Posterior to the postcentral sulcus there is a large area, subdivided by the intraparietal sulcus. It usually starts in the postcentral sulcus near its midpoint and extends posteroinferiorly across the parietal lobe, dividing it into superior and inferior parietal lobules. Posteriorly, its occipital ramus extends into the occipital lobe, joining the transverse occipital sulcus at right angles.
Somatosensory Cortex
The primary somatosensory cortex contains within it a topographical map of the contralateral half of the body. The face, tongue and lips are represented inferiorly; the trunk and upper limbs are represented on the superolateral aspect and the lower limbs on the medial aspect of the hemisphere, giving rise to the familiar ‘homunculus’ map (see Fig. 16.13).
The somatosensory properties of SI depend on its thalamic input from the ventral posterior nucleus of the thalamus, which in turn receives the medial lemniscal, spinothalamic and trigeminothalamic pathways. The nucleus is divided into a ventral posterolateral part, which receives information from the trunk and limbs, and a ventral posteromedial part, in which the head is represented. Within the ventral posterior nucleus, neurones in the central core respond to cutaneous stimuli, and those in the most dorsal anterior and posterior parts, which arch as a ‘shell’ over this central core, respond to deep stimuli. This is reflected in the differential projections to SI: the cutaneous central core projects to 3b, the deep tissue–responsive neurones send fibres to areas 3a and 2 and an intervening zone projects to area 1. Within the ventral posterior nucleus, anteroposterior rods of cells respond with similar modality and somatotopic properties. They appear to project to restricted focal patches in SI of approximately 0.5 mm, which form narrow strips mediolaterally along SI. The laminar termination of thalamocortical axons from the ventral posterior nucleus is different in the separate cytoarchitectonic subdivisions of SI. In areas 3a and 3b these axons terminate mainly in layer IV and the adjacent deep part of layer III, whereas in areas 1 and 2 they end in the deeper half of layer III, avoiding lamina IV. Additional thalamocortical fibres to SI arise from the intralaminar system, notably the centrolateral nucleus.
Superior and Inferior Parietal Lobules
Posterior to the postcentral gyrus, the superior part of the parietal lobe is composed of areas 5, 7a and 7b (see Fig. 16.12; Fig. 16.16). Area 5 receives a dense feed-forward projection from all cytoarchitectonic areas of SI in a topographically organized manner. The thalamic afferents to this area come from the lateral posterior nucleus and from the central lateral nucleus of the intralaminar group. Ipsilateral corticocortical fibres from area 5 go to area 7, the premotor and supplementary motor cortices, the posterior cingulate gyrus and the insular granular cortex. Commissural connections between area 5 on both sides tend to avoid the areas of representation of the distal limbs. The response properties of cells in area 5 are more complex than in SI, with larger receptive fields and evidence of submodality convergence. Area 5 contributes to the corticospinal tract.
In monkeys, area 7a is not related to the cortical pathways for somatosensory processing; instead, it forms part of a dorsal cortical pathway for spatial vision. The major ipsilateral corticocortical connections to area 7a are derived from visual areas in the occipital and temporal lobes. In the ipsilateral hemisphere, area 7a has connections with the posterior cingulate cortex (area 24) and with areas 8 and 46 of the frontal lobe. Commissural connections are with its contralateral homologue. Area 7a is connected with the medial pulvinar and intralaminar paracentral nuclei of the thalamus. In experimental studies, neurones within area 7a are visually responsive. They relate largely to peripheral vision, respond to stimulus movement and are modulated by eye movement.
Injury of the superior parietal cortex in humans can lead to the inability to recognize the shapes of objects by touch (astereognosis) and a variety of disorders reflecting breakdown of the body scheme or body image, such as difficulty assimilating spatial perception of the body (amorphosynthesis) and sensory neglect of the contralateral body (asomatognosia), which causes a variety of syndromes, including so-called dressing apraxia (see Case 3). More complex perceptional disturbances follow damage of the inferior parietal cortex, including areas 39 and 40. These include difficulties with language, because Wernicke’s speech area includes parts of the inferior parietal lobe of the dominant hemisphere (see Fig. 16.16 and Case 12), and dyscalculia if the dominant hemisphere is involved. Contralateral sensory neglect extends to the extracorporeal space and includes the visual appreciation of the world, such as the omission of one side (usually the left) of a drawing when a patient is asked to copy a sketch of a clock face. Difficulties with complex orientation in space, such as map reading, are also seen.
Temporal Lobe
Thus, the lateral surface is divided into three parallel gyri: superior (area 22), middle (area 21) and inferior (area 20) temporal gyri. The temporal pole (area 38) lies in front of the termination of these gyri. Along its superior margin, the superior temporal gyrus is continuous with gyri in the floor of the posterior ramus of the lateral sulcus. These vary in number and extend obliquely anterolaterally from the circular sulcus around the insula as transverse temporal gyri of Heschl (Fig. 16.17). The anterior transverse temporal gyrus and adjoining part of the superior temporal gyrus are auditory in function and are considered to be Brodmann’s area 42. The anterior gyrus is approximately area 41.
Auditory Cortex
The temporal operculum houses the primary auditory cortex, AI (Fig. 16.18). This is coextensive with granular area 41 in the transverse temporal gyri. Surrounding areas constitute auditory association cortex. The primary auditory cortex is reciprocally connected with all subdivisions of the medial geniculate nucleus and may receive additional thalamocortical projections from the medial pulvinar. The geniculocortical fibres terminate densely in layer IV. AI contains a tonotopic representation of the cochlea in which high frequencies are represented posteriorly and low frequencies anteriorly. Single-cell responses are to single tones of a narrow frequency band. Cells in single vertical electrode penetrations share an optimal frequency response.
Insula
The insula lies deep in the floor of the lateral fissure; it is almost surrounded by a circular sulcus and is overlapped by adjacent cortical areas, the opercula (see Figs 16.17–16.20). The frontal operculum is between the anterior and ascending rami of the lateral fissure, forming a triangular division of the inferior frontal gyrus. The frontoparietal operculum, between ascending and posterior rami of the lateral fissure, consists of the posterior part of the inferior frontal gyrus, the lower ends of the precentral and postcentral gyri and the lower end of the anterior part of the inferior parietal lobule. The temporal operculum, below the posterior ramus of the lateral fissure, is formed by superior temporal and transverse temporal gyri. Anteriorly, the inferior region of the insula adjoins the orbital part of the inferior frontal gyrus.
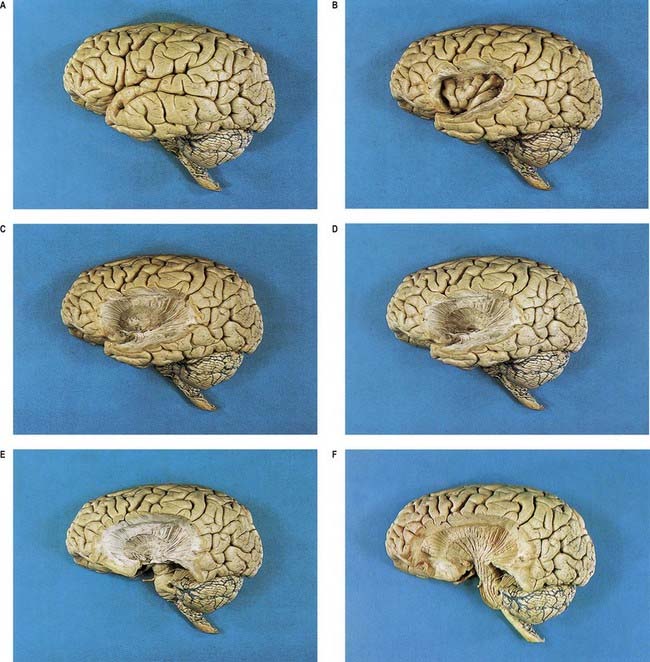
Fig. 16.20 Series of dissections of the left cerebral hemisphere at progressively deeper levels to demonstrate the insula and subjacent structures. A, Intact brain. B, Cortical gyri of the insula exposed by removal of the frontal, temporal and parietal opercula. C, Removal of the insular cortex, extreme capsule, claustrum and external capsule to expose the lateral aspect of the putamen. D, Removal of the lentiform complex to display fibres of the internal capsule. E, Removal of part of the temporal lobe to show the internal capsule fibres converging on the crus cerebri of the midbrain. F, Removal of the optic tract and superficial dissection of the pons and upper medulla, emphasizing the continuity of the corona radiata, internal capsule, crus cerebri, longitudinal pontine fibres and medullary pyramid.
(Dissections by E. L. Rees; photographs by Kevin Fitzpatrick on behalf of GKT School of Medicine, London.)
When the opercula are removed, the insula appears as a pyramidal area, its apex beneath and near the anterior perforated substance, where the circular sulcus is deficient (see Figs 16.19, 16.20). The medial part of the apex is termed the limen insulae (gyrus ambiens). A central insular sulcus, which slants posterosuperiorly from the apex, divides the insular surface into a large anterior part and a small posterior part. The anterior part is divided by shallow sulci into three or four short gyri, whereas the posterior part is one long gyrus, often divided at its upper end. The cortex of the insula is continuous with that of its opercula in the circular sulcus. The insula is approximately coextensive with the subjacent claustrum and putamen.
Claustrum
The claustrum (see Figs 16.35, 16.42) is a thin sheet of grey matter lying deep to the insula. It is approximately coextensive with the insula, from which it is separated by the extreme capsule. Medially, the claustrum is separated from the putamen by the external capsule. It is thickest anteriorly and inferiorly, where it becomes continuous with the anterior perforated substance, amygdala and prepiriform cortex. In animals, it has reciprocal, topographically organized connections with many regions of the neocortex. Little is known about the connections and functional significance of the claustrum in the human brain.
Occipital Lobe
The occipital lobe lies behind an arbitrary line joining the preoccipital incisure and the parieto-occipital sulcus. The transverse occipital sulcus descends from the superomedial margin of the hemisphere, behind the parieto-occipital sulcus, and is joined about its midpoint by the intraparietal sulcus. The lateral occipital sulcus divides the lobe into superior and inferior occipital gyri (see Figs 16.1, 16.2). The lunate sulcus, when present, lies just in front of the occipital pole. It is placed vertically and is occasionally joined to the calcarine sulcus. Its lips separate striate from peristriate areas; the parastriate area is buried in the sulcus between the other two striate areas. The lunate sulcus is posterior to the gyrus descendens, which is behind the superior and inferior occipital gyri. Curved superior and inferior polar sulci often appear near the ends of the lunate sulcus. The superior polar sulcus arches up onto the medial occipital surface near the upper limit of the lunate sulcus. The inferior polar sulcus arches down and forward onto the inferior cerebral surface from the lower limit of the lunate sulcus. These polar sulci enclose semilunar extensions of the striate area and indicate the extent of the visual cortex associated with the macula.
The primary visual cortex is located mostly on the medial aspect of the occipital lobe and is coextensive with the subcortical nerve fibre stria of Gennari in layer IV; hence, its alternative name, the striate cortex. It occupies the upper and lower lips and depths of the posterior part of the calcarine sulcus and extends into the cuneus and lingual gyrus (Fig. 16.21). Posteriorly, it is limited by the lunate sulcus and by polar sulci above and below this sulcus. It extends to the occipital pole.
The primary visual cortex receives afferent fibres from the lateral geniculate nucleus (see Figs 15.7, 15.8) via the optic radiation. The latter curves posteriorly and spreads through the white matter of the occipital lobe. Its fibres terminate in strict point-to-point fashion in the striate area. The cortex of each hemisphere receives impulses from two half retinae, which represent the contralateral half of the binocular visual field. Superior and inferior retinal quadrants are connected with corresponding areas of the striate cortex. Thus, the superior retinal quadrants (representing the inferior half of the visual field) are connected with the visual cortex above the calcarine sulcus, and the inferior retinal quadrants (representing the upper half of the visual field) are connected with the visual cortex below the calcarine sulcus. The peripheral parts of the retinae activate the most anterior parts in the visual cortex. The macula impinges on a disproportionately large posterior part.
The striate cortex is granular. Layer IV, bearing the stria of Gennari, is commonly divided into three sublayers. Passing from superficial to deep, these are IVA, IVB (which contains the stria), and IVC. The densely cellular IVC is further subdivided into a superficial IVCα and a deep IVCβ. Layer IVB contains only sparse, mainly non-pyramidal neurones. The input to area 17 from the lateral geniculate nucleus terminates predominantly in layers IVA and IVC. Other thalamic afferents, from the inferior pulvinar nucleus and the intralaminar group, pass to layers I and VI. Geniculocortical fibres terminate in alternating bands. Axons from geniculate laminae, which receive information from the ipsilateral eye (laminae II, III and V), are segregated from those of laminae receiving input from the contralateral eye (laminae I, IV and VI). Neurones within layer IVC are monocular; that is, they respond to stimulation of either the ipsilateral or contralateral eye, but not both. This horizontal segregation forms the anatomical basis of the ocular dominance column, in that neurones encountered in a vertical strip from pia to white matter exhibit a preference for stimulation of one eye or the other, even though they are binocular outside layer IV. The other major functional basis for the columnar organization of the visual cortex is the orientation column: that is, an electrode passing through the depth of the cortex at right angles to the plane from pia to white matter encounters neurones that all respond preferentially to either a stationary or a moving straight line of a given orientation within the visual field. Cells with simple, complex and hypercomplex receptive fields occur in area 17. Simple cells respond optimally to lines in a narrowly defined position. Complex cells respond to a line anywhere within a receptive field, but with a specific orientation. Hypercomplex cells are similar to complex cells, except that the length of the line or bar stimulus is critical for an optimal response. There is a relationship between the complexity of response and the position of cells in relation to the cortical laminae. Simple cells are mainly in layer IV, and complex and hypercomplex cells predominate in either layers II and III or layers V and VI.
Limbic Lobe
The limbic lobe includes large parts of the cortex on the medial wall of the hemisphere, principally the subcallosal, cingulate and parahippocampal gyri (Fig. 16.22). It also includes the hippocampal formation, which consists of the hippocampus proper (Ammon’s horn or cornu ammonis), the dentate gyrus, the subicular complex (subiculum, presubiculum, parasubiculum) and the entorhinal cortex (area 28). There is a close relationship between these phylogenetically old cortical structures and the termination of the olfactory tract in the frontal and medial temporal lobes.
On the basis of emotional disturbances displayed by patients who presented with damage to the hippocampus and cingulate gyrus, Papez (1937) described a closed circuit (the Papez circuit) that links the hippocampus with the cingulate cortex, via the mammillary bodies and anterior thalamus. He proposed that emotional expression is organized in the hippocampus, experienced in the cingulate gyrus and expressed via the mammillary bodies. The hypothalamus was thought to be the site where hippocampal processes gain access to the autonomic outflow that controls the peripheral expression of emotional states. The Papez circuit is now widely accepted as being involved with cognitive processes, including mnemonic functions and spatial short-term memory.
The cingulate gyrus is the area that shows the most consistent pain-evoked changes in synaptic activity related to regional cerebral bloodflow as measured by either positron emission tomography (PET) or functional magnetic resonance imaging (fMRI) (Derbyshire et al 1997). It must be remembered that pain evokes a multidimensional response in the brain, including cognitive, emotional, autonomic and motor components, so it is not always possible to assign specific functions to parts of the brain that generate PET or fMRI signals in response to pain Peyron, Laurent and Garcia-Larrea 2000). However, in many experimental paradigms, a combination of signals from the cingulate gyrus, somatosensory area SII and insula appears to be involved in the conscious appreciation of nociception and neuropathic pain.
The complex parahippocampal gyrus includes areas 27, 28 (entorhinal cortex), 35, 36, 48 and 49 and temporal cortical fields. The rich interconnections within the cingulate and parahippocampal cortices, and with the hippocampal formation, are schematically represented in Figure 16.23. Only a few are described in detail here. In monkeys, the infralimbic cortex (area 25) has been shown to project to areas 24a and 24b. Area 25 also has reciprocal connections with the entorhinal cortex. Projections between paralimbic area 32 and the limbic cortex (anterior, retrosplenial and entorhinal cortex) are somewhat less prominent. Areas 24 and 29 are connected with the paralimbic posterior cingulate area 23. The strong connections between subicular and entorhinal areas are discussed in the context of the hippocampal formation itself. Reference to Figure 16.23 emphasizes the way the pro-isocortical cingulate and related areas (32, 24c, 23, 29d, 35b, 36) interface between the limbic-archicortex and peri-archicortex and widespread areas of the neocortex. This pattern of cortical connection—outward from the hippocampus via the entorhinal cortex to the perirhinal cortex, caudal parahippocampal gyrus and posterior cingulate gyrus—has enormous functional importance as far as the hippocampus is concerned, as discussed later. The parahippocampal gyrus projects to virtually all association areas of the cortex in primates and also provides the major funnel through which polymodal sensory inputs converge on the hippocampus.
CASE 10 Herpes Encephalitis
A 26-year-old woman is brought to the emergency room because of agitation and bizarre behaviour of 3 days’ duration. She previously complained of malaise and headache and has a history of anxiety and depression. Her general physical examination is normal. She makes occasional inappropriate, sexually explicit comments during the examination, as well as provocative sexually oriented displays. She remains agitated, with repeated emotional outbursts, and ultimately needs to be restrained. There are no focal neurological deficits on examination.
Discussion: Herpes encephalitis is characterized by behavioural and personality changes. Aphasia may be present. The clinical syndrome may resemble the changes encountered in the Klüver-Bucy experimental model following bilateral temporal lobectomy. Anatomically, medial temporal structures are most consistently involved (Fig. 16.24). Lesions may be more widespread, however, involving orbital frontal and cingulate gyri. Many patients exhibit a marked memory deficit as a residual finding.
Olfactory Pathways
The olfactory pathways include the olfactory nerves, olfactory bulb and olfactory tract, together with its central connections in the frontal and temporal lobes. These are primarily described in Chapter 12. The close association of these structures with limbic regions is shown in Figure 16.7.
Hippocampal Formation
The hippocampus lies above the subiculum and medial parahippocampal gyrus, forming a curved elevation, approximately 5 cm long, along the floor of the inferior horn of the lateral ventricle (Fig. 16.25). Its anterior end is expanded, and its margin there may have two or three shallow grooves that give it a paw-like appearance, the pes hippocampi. The ventricular aspect is convex. It is covered by ependyma, beneath which fibres of the alveus converge medially on a longitudinal bundle of fibres, the fimbria of the fornix (Fig. 16.26). Passing medially from the collateral sulcus, the neocortex of the parahippocampal gyrus merges with the transitional juxtallocortex of the subiculum. The latter curves superomedially to the inferior surface of the dentate gyrus, then laterally to the laminae of the hippocampus. The curvature continues, first superiorly, then medially above the dentate gyrus, and ends pointing toward the centre of the superior surface of the dentate gyrus. The dentate gyrus is a crenated strip of cortex related inferiorly to the subiculum, laterally to the hippocampus and, more medially, to the fimbria of the fornix (Figs 16.26, 16.27). The form of the fimbria is quite variable, but medially, it is separated from the crenated medial margin of the dentate gyrus by the fimbriodentate sulcus (Fig. 16.28). The hippocampal sulcus, of variable depth, lies between the dentate gyrus and the subicular extension of the parahippocampal gyrus. Posteriorly, the dentate gyrus is continuous with the gyrus fasciolaris and thus with the indusium griseum. Anteriorly, it continues into the notch of the uncus, turning medially across its inferior surface, as the tail of the dentate gyrus (band of Giacomini) and vanishes on the medial aspect of the uncus (see Fig. 16.27). The tail separates the inferior surface of the uncus into an anterior uncinate gyrus and posterior intralimbic gyrus.
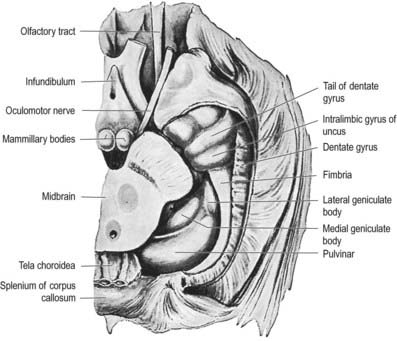
Fig. 16.27 Basal aspect of the brain dissected to display the dentate gyrus, uncus and fimbria on the left.
The hippocampus is trilaminar archicortex. It consists of a single pyramidal cell layer, with plexiform layers above and below it. It can be divided into three distinct fields: CA1, CA2 and CA3 (Figs 16.28, 16.29). Field CA3 borders the hilus of the dentate gyrus at one end, and field CA2 at the other. Field CA3 pyramidal cells are the largest in the hippocampus, and the whole pyramidal cell layer in this field is about 10 cells thick. The most important feature of pyramidal cells in CA3 is that they receive the mossy fibre input from dentate granule cells on their proximal dendrites. The border between CA3 and CA2 is not well marked because the pyramidal cells of the former appear to extend under the border of the latter for some distance. The CA2 field has the most compact layer of pyramidal cells. It completely lacks a mossy fibre input from dentate granule cells and receives a major input from the supramammillary region of the hypothalamus. Field CA1 is usually described as the most complex of the hippocampal subdivisions, and its appearance varies along its transverse and rostrocaudal axes. The CA1–CA2 border is not sharp, and at its other end, CA1 overlaps the subiculum for some distance. The thickness of the pyramidal cell layer varies from 10 to more than 30 cells. Approximately 10% of neurones in this field are interneurones.
It is common to describe several strata within the layers of the hippocampus (see Figs 16.28, 16.29). Starting from the ventricular aspect, these are the alveus (containing subicular and hippocampal pyramidal cell axons converging on the fimbria of the fornix), stratum oriens (mainly the basal dendrites of pyramidal cells and some interneurones), stratum pyramidalis, stratum lucidum (containing mossy fibres that make contact with the proximal dendrites of pyramidal cells in field CA3), stratum radiatum and stratum lacunosum-moleculare. The stratum lucidum is not as prominent in humans as it is in other primates, and it is not present in fields CA1 and CA2.
The subicular complex is generally subdivided into the subiculum, presubiculum and parasubiculum (see Figs 16.28, 16.29). The major subcortical projections of the hippocampal formation (to the septal nuclei, mammillary nuclei, nucleus accumbens and anterior thalamus), and those to the entorhinal cortex, all arise from pyramidal neurones of the subicular complex. The subiculum consists of a superficial molecular layer containing apical dendrites of subicular pyramidal cells, a pyramidal cell layer that is approximately 30 cells thick and a deep polymorphic layer. The presubiculum is medial to the subiculum and is distinguished by a densely packed superficial layer of pyramidal cells. There is a plexiform layer superficial to this dense cell layer. Cells deep to it are regarded as either a medial extension of the subiculum or a lateral extension of the deep layers of the entorhinal cortex. The parasubiculum also has a superficial plexiform layer and a primary cell layer. It forms the boundary between the subicular complex as a whole and the entorhinal cortex. The cell layers deep to the parasubiculum are indistinguishable from the deep layers of the entorhinal cortex.
The entorhinal cortex (Brodmann’s area 28; see Figs 16.7, 16.28, 16.29) extends rostrally to the anterior limit of the amygdala. Caudally, it overlaps a portion of the hippocampal fields. The more primitive levels of the entorhinal cortex (below the amygdala) receive projections from the olfactory bulb. More caudal regions do not generally receive primary olfactory inputs.
Glutamate or aspartate appears to be the major excitatory transmitter in three pathways in the hippocampal formation—namely, the perforant pathway, which arises in the entorhinal cortex and terminates primarily in the dentate gyrus; the mossy fibres, which run from the dentate granule cells to the pyramidal cells of the CA3 field; and the Schaffer collaterals of CA3 pyramidal cells, which terminate on CA1 pyramidal cells.
The subiculum, rather than the hippocampus, projects to the mammillary complex, whereas the hippocampus gives rise principally to efferents destined for the septal complex. Summaries of hippocampal circuitry and connections are shown schematically in Figure 16.30.
In humans, the fornix contains approximately 1.2 million fibres. Cells in the CA3 field project bilaterally to the lateral nucleus of the septal complex, via the precommissural fornix. They give rise to the Schaffer collaterals to CA1 cells and to the commissural projections to the contralateral hippocampus. Neurones in the subicular complex and entorhinal cortex give rise to projections to the nucleus accumbens and to parts of the caudate nucleus and putamen. The subicular complex gives rise to the major postcommissural fibre system of the fornix. The presubiculum, in particular, projects to the anterior thalamic nuclear complex (anteromedial, anteroventral and laterodorsal nuclei). Both the subiculum and the presubiculum provide the major extrinsic input to the mammillary complex. Both the lateral and the medial mammillary nuclei receive afferents from the subicular complex.
CASE 11 Alzheimer’s Disease
Discussion: This man demonstrates the typical devastating, progressive clinical abnormalities of Alzheimer’s disease, probably the most common dementing illness of middle and later life (to be distinguished from diffuse Lewy body disease, vascular dementia, and normal-pressure hydrocephalus, for example). Ultimately, much of the cerebral grey matter is involved, with typical neuropathological alterations and neurofibrillary changes reflecting intracellular accumulation of the protein tau, amyloid-containing senile plaques, deposition of amyloid in small vessel walls and neuronal vacuolization and loss. The earliest morphological changes are found in the medial temporal lobe and in the basal nucleus (of Meynert), with secondary loss of acetylcholine transferase, especially in the neocortex, reflecting the degeneration of cholinergic projections from the basal nucleus (Fig. 16.31).
Septum
The septum is a midline and paramedian structure (see Figs 14.6, 16.22). Its upper portion corresponds largely to the bilateral laminae of fibres, sparse grey matter and neuroglia known as the septum pellucidum, which separates the lateral ventricles. Below this, the septal region is made up of four main nuclear groups: dorsal, ventral, medial and caudal. The dorsal group is essentially the dorsal septal nucleus, the ventral group consists of the lateral septal nucleus, the medial group contains the medial septal nucleus and the nucleus of the diagonal band of Broca and the caudal group contains the fimbrial and triangular septal nuclei.
Amygdala
The amygdaloid nuclear complex is made up of lateral, central and basal nuclei that lie in the dorsomedial temporal pole, anterior to the hippocampus, and close to the tail of the caudate nucleus (see Fig. 16.22). Collectively, the nuclei form the ventral, superior and medial walls of the tip of the inferior horn of the lateral ventricle. The amygdala is partly continuous above with the inferomedial margin of the claustrum. Fibres of the external capsule and substriatal grey matter, including the cholinergic magnocellular nucleus basalis (of Meynert), incompletely separate it from the putamen and globus pallidus. Laterally, it is close to the optic tract. It is partly deep to the gyrus semilunaris, gyrus ambiens and uncinate gyrus (see Fig. 16.7).
It has been suggested that the basolateral complex of nuclei (lateral, basal, accessory basal) shares several characteristics with the cortex and that it may be considered a quasi-cortical structure. Although it lacks a laminar structure, it has direct, often reciprocal, connections with adjacent temporal and other areas of cortex, and it projects to the motor or premotor cortex. It receives a direct cholinergic and non-cholinergic input from the magnocellular corticopetal system in the basal forebrain and has reciprocal connections with the mediodorsal thalamus. The distribution of small peptidergic neurones in the basolateral nuclear complex (e.g. those containing neuropeptide Y, somatostatin and CCK) are also similar in form and density to those found in the adjacent temporal lobe cortex. Projection neurones from this part of the amygdala appear to utilize, at least in part, the excitatory amino acids glutamate and aspartate as transmitters. Moreover, they project to the ventral striatum rather than to hypothalamic and brain stem sites. Thus, it may be appropriate to consider this part of the amygdaloid complex as a polymodal cortex-like area that is separated from the cerebral cortex by fibres of the external capsule.
The central nucleus is present through the caudal half of the amygdaloid complex, lying dorsomedial to the basal nucleus. It is divided into medial and lateral parts. The medial part, which contains larger cells than the lateral part, resembles the adjacent putamen. The medial and central nuclei appear to have an extension across the basal forebrain, as well as within the stria terminalis, which merges with the bed nucleus of the stria terminalis. This extensive nuclear complex, sometimes referred to as the ‘extended amygdala,’ is illustrated in Figure 16.32. It can be considered a macrostructure formed by the centromedial amygdaloid complex (medial nucleus, medial and lateral parts of the central nucleus), the medial bed nucleus of the stria terminalis and the cell columns that traverse the sublenticular substantia innominata, which lies between them. It has been suggested that portions of the medial nucleus accumbens may be included in the extended amygdala.
Efferent Connections
The central nucleus is the major relay for amygdaloid projections to the hypothalamus. Amygdaloid fibres reach the bed nucleus of the stria terminalis primarily via the stria terminalis, but also via the ventral amygdalofugal pathway. In general, central and basal nuclei project to the lateral part of the bed nucleus, whereas medial and posterior cortical nuclei project to the medial bed nucleus. Anterior cortical and medial nuclei project largely to the medial preoptic area and anterior medial hypothalamus, including the paraventricular and supraoptic nuclei. There is a particularly prominent projection to the ventromedial and premammillary nuclei. The amygdala projects to the rostrocaudal extent of the lateral hypothalamus. The majority of the fibres originate in the central nucleus and run principally in the ventral amygdalofugal pathway and medial forebrain bundle.
White Matter of Cerebral Hemisphere
Association Fibres
Association fibres may be either short association (arcuate or U) fibres, which link adjacent gyri, or long association fibres, which connect more widely separated gyri (Figs 16.33, 16.34). Short association fibres may be entirely intracortical. Many pass subcortically between adjacent gyri; some merely pass from one wall of a sulcus to the other.
CASE 12 Aphasias
A 49-year-old man with hypertrophic cardiomyopathy has three episodes of difficulty speaking over the course of a day. The episodes are brief, each lasting less than 30 seconds. He is fully aware of the events. He is able to understand what is being said to him at the time. The following day he loses consciousness and is taken to the local hospital, where he is found to have an irregular cardiac rhythm. He attempts to speak but is unable to produce any words or sounds. He is able to follow both written and verbal commands. Brain MRI reveals an acute infarct in the left inferior frontal lobe. The patient is diagnosed with Broca’s aphasia secondary to a stroke caused by a cardiac embolism. Over the course of several weeks, he gradually regains the ability to speak; however, his fluency and ability to repeat phrases spoken to him remain impaired.
Commissural Fibres
Corpus Callosum
The corpus callosum is the largest fibre pathway of the brain (Figs. 16.2, 16.4, 16.35, 16.36). It links the cerebral cortex of the two cerebral hemispheres, and it roofs much of the lateral ventricles. It forms an arch approximately 10 cm long, with an anterior end approximately 4 cm from the frontal poles and a posterior end approximately 6 cm from the occipital poles. Its anterior portion is known as the genu. This recurves posteroinferiorly in front of the septum pellucidum, then diminishes rapidly in thickness and is prolonged to the upper end of the lamina terminalis as the rostrum. The trunk of the corpus callosum arches back and is convex above. It ends posteriorly in the expanded splenium, which is its thickest part.
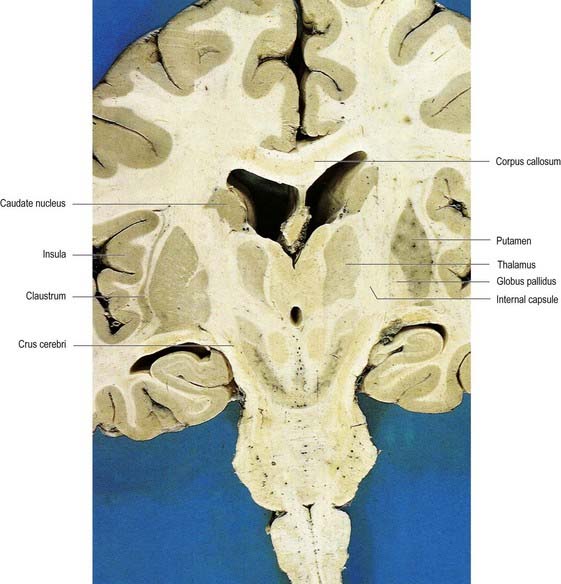
Fig. 16.35 Oblique coronal section of the brain.
(Dissection by E. L. Rees; photograph by Kevin Fitzpatrick on behalf of GKT School of Medicine, London.)
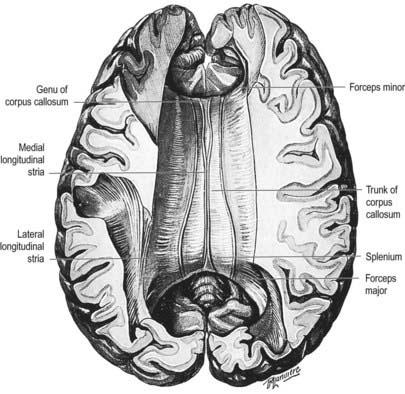
Fig. 16.36 Superior aspect of the corpus callosum revealed by partial removal of the cerebral hemispheres.
The superior surface of the callosal trunk (see Fig. 16.35) is covered by a thin layer of grey matter, the indusium griseum. This extends anteriorly around the genu; then, on the inferior aspect of the rostrum, it continues into the paraterminal gyrus. It contains narrow longitudinal bundles of fibres on each side, the medial and lateral longitudinal striae. Posteriorly, the indusium griseum is continuous with the dentate gyrus and hippocampus through the gyrus fasciolaris (Fig. 16.37).
Nerve fibres of the corpus callosum radiate into the white matter core of each hemisphere, thereafter dispersing to the cerebral cortex. Commissural fibres forming the rostrum extend laterally, below the anterior horn of the lateral ventricle, connecting the orbital surfaces of the frontal lobes. Fibres in the genu curve forward, as the forceps minor, to connect the lateral and medial surfaces of the frontal lobes. Fibres of the trunk pass laterally, intersecting with the projection fibres of the corona radiata to connect wide neocortical areas of the hemispheres (Figs 16.38, 16.39). Fibres of the trunk and splenium, which form the roof and lateral wall of the posterior horn and the lateral wall of the inferior horn of the lateral ventricle, constitute the tapetum. The remaining fibres of the splenium curve back into the occipital lobes as the forceps major.
Congenital absence of the corpus callosum is rare. When it occurs, the clinical history usually lacks diagnostic features. This is perhaps not surprising, because apparently little disturbance of function occurs when large parts, and in some cases all, of the corpus callosum are surgically divided for the control of intractable epilepsy. The studies of Sperry (1974, 1984) on the effects of dividing the human corpus callosum revealed its function in the transfer of information (including memorized data) across the midline of the cerebrum and confirmed a long-suspected asymmetry of function, leading to the concept of ‘dominance,’ usually by the left hemisphere.
CASE 13 Glioma of the Corpus Callosum
Discussion: Glioma of the corpus callosum, particularly early in its course, must be distinguished from Alzheimer’s disease, multi-infarct dementia, frontotemporal dementia, Parkinson’s disease, and late-onset depression, as well as a host of systemic disorders. Neuroimaging is clearly diagnostic in these circumstances (Fig. 16.40). The tumour may be found in the genu, body, or splenum of the corpus callosum.
CASE 14 Marchiafava–Bignami Disease
An elderly alcoholic becomes confused, exhibiting a progressive dementing illness with occasional seizures and a mixture of multifocal (pyramidal and extrapyramidal) clinical signs. The disorder evolves over several months, with occasional complete or incomplete remissions marking its overall course. Necrosis of the mesial portion of the corpus callosum is observed with MRI, amply confirmed on postmortem examination. The lesion in the corpus callosum involves especially its anterior part, and frank cavitation can be seen. The anterior commissure and occasionally other commissural bundles may be similarly involved, and lesions may spread from the corpus callosum into the centrum semiovale bilaterally (Fig. 16.41).
Anterior Commissure
The anterior commissure is a compact bundle of myelinated nerve fibres that crosses anterior to the columns of the fornix and is embedded in the lamina terminalis, where it is part of the anterior wall of the third ventricle (see Fig. 16.22). In sagittal section it is oval; its long (vertical) diameter is approximately 1.5 mm. Laterally, it splits into two bundles. The smaller anterior bundle curves forward on each side to the anterior perforated substance and olfactory tract. The posterior bundle curves posterolaterally on each side in a deep groove on the anteroinferior aspect of the lentiform complex and subsequently fans out into the anterior part of the temporal lobe, including the parahippocampal gyrus. Areas thought to be connected via commissural fibres include the olfactory bulb and anterior olfactory nucleus; the anterior perforated substance, olfactory tubercle and diagonal band of Broca; the prepiriform cortex; the entorhinal area and adjacent parts of the parahippocampal gyrus; part of the amygdaloid complex, especially the nucleus of the lateral olfactory stria; the bed nucleus of the stria terminalis and the nucleus accumbens; and the anterior regions of the middle and inferior temporal gyri.
Projection Fibres
Projection fibres connect the cerebral cortex with lower levels in the brain and spinal cord. They include large numbers of both corticofugal and corticopetal projections. Corticofugal projection fibres converge from all directions to form the dense subcortical white matter mass of the corona radiata. Large numbers of fibres pass to the corpus striatum and the thalamus, intersecting commissural fibres of the corpus callosum en route (see Fig. 16.39). The corona radiata is continuous with the internal capsule, which contains the majority of the cortical projection fibres.
Internal Capsule
In horizontal cerebral sections, the internal capsule appears as a broad white band, with a lateral concavity, that accommodates the lentiform complex (see Figs 16.35, 16.38; Figs 16.42–16.44). It has an anterior limb, genu, posterior limb, and retrolenticular (retrolentiform) and sublenticular (sublentiform) parts. Both anterior and posterior limbs are medial to the lentiform complex. The head of the caudate nucleus is medial to the anterior limb, and the thalamus is medial to the posterior limb. Cortical efferent fibres of the internal capsule continue to converge as they descend. Fibres derived from the frontal lobe tend to pass posteromedially, whereas temporal and occipital fibres pass anterolaterally. Many, but not all, corticofugal fibres pass into the crus cerebri of the ventral midbrain. There, corticospinal and corticobulbar fibres are located in the middle half of the crus. Frontopontine fibres are located medially, whereas corticopontine fibres from temporal, parietal and occipital cortices are found laterally.
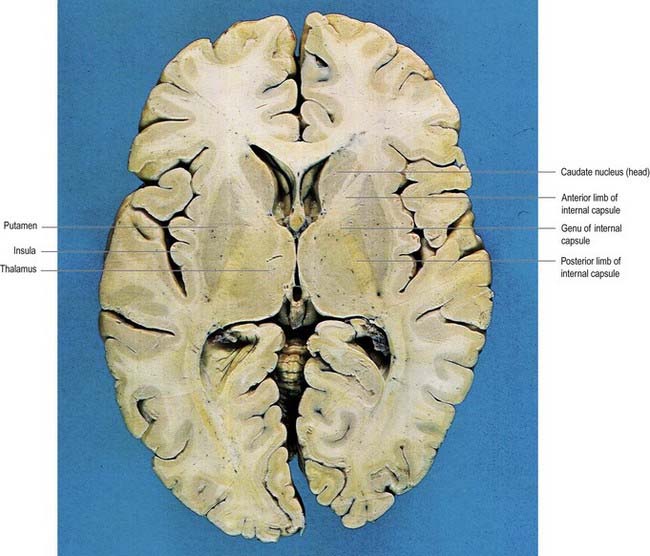
Fig. 16.43 Horizontal section of the brain through the frontal and occipital poles of the cerebral hemispheres.
(Dissection by E. L. Rees; photograph by Kevin Fitzpatrick on behalf of GKT School of Medicine, London.)
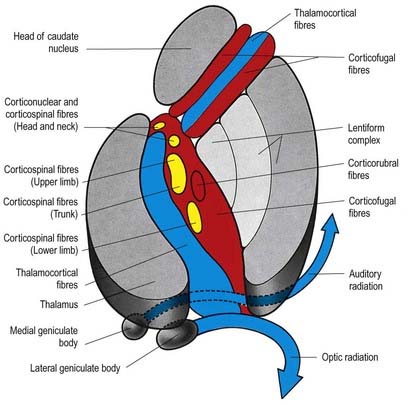
Fig. 16.44 Horizontal section through the internal capsule illustrating its main fibre components. Descending motor fibres, yellow; corticofugal fibres to the thalamus and pons, red; ascending fibres, blue.
(From Truex, R.C. [Ed.], Strong and Elwyn’s Human Neuroanatomy, 4th ed. London: Baillière Tindall and Cox, London; and Kretschmann, H.-J., 1998. Localization of the corticospinal fibres in the internal capsule in man. J. Anat. [Lond.] 160, 219–225.)
The optic radiation arises in the lateral geniculate body. It sweeps backward, intimately related to the superolateral aspect of the inferior horn and the lateral aspect of the posterior horn of the lateral ventricle, from which it is separated by the tapetum.
Cerebral Asymmetry
The two human cerebral hemispheres are not simply mirror images of each other. In 1861, Broca described a case of expressive aphasia resulting from an infarct in the left posterior inferior frontal lobe, which became known as Broca’s area. The later discovery of Wernicke’s area in the left posterior temporal and inferior parietal lobes provided unequivocal evidence of another lateralized function and demonstrated an asymmetry for language comprehension as well as for speech production. The association of language impairment with left hemisphere lesions led to the more general concept of a dominant left hemisphere and a minor right hemisphere.
Much information on the lateralization of cerebral function has come from studying patients in whom the corpus callosum was divided (commissurotomy) to treat intractable epilepsy and rare subjects who lack part or all of the corpus callosum. Commissurotomy produces the ‘split-brain’ syndrome (Fig. 16.45), supporting the notion that abilities or functions are predominantly associated with one hemisphere or the other. Knowledge of such lateralization of function has been advanced more recently by functional brain imaging techniques such as PET.
Certain cerebral anatomical asymmetries are apparent at both the macroscopic and histological levels. One of the most notable is in the planum temporale, which is usually larger on the left side than the right (Fig. 16.46). Probably as a result of this size difference, the lateral fissure is longer and more horizontal in the left hemisphere; this observation, together with the orientation of the overlying vasculature, provides a surface marker of temporal lobe asymmetry. The limits of asymmetry in the superior temporal lobe remain uncertain but appear to include Heschl’s gyrus and some other structures adjacent to (and sometimes considered an extension of) the planum temporale.
There is evidence that planum temporale asymmetry originates almost entirely from right–left differences in the size of a cytoarchitectonic subfield called Tpt (see Fig. 16.46). Subtle asymmetries in the superior temporal lobe have been demonstrated in terms of overall size and shape, sulcal pattern and cytoarchitecture, as well as at the neuronal level. It seems reasonable to assume that these differences underlie some of the functional asymmetry for language representation.
CASE 15 Creutzfeldt-Jakob Disease
Discussion: The combination of history, neurological examination and diagnostic test results points strongly to a diagnosis of Creutzfeldt-Jakob disease, a human prion disease and one of the so-called transmissible spongiform encephalopathies. Anatomically, the disorder involves grey matter diffusely throughout the neuraxis, with remarkable devastation, especially of the cerebral cortex, but ultimately involving the entire central nervous system (Fig. 16.47).
Alheid, Heimer, 1988Alheid G.F. Heimer L. New perspectives in basal forebrain organization of special relevance for neuropsychiatric disorders: the striatopallidal, amygdaloid and corticopetal components of the substantia innominata. Neuroscience. 27:1988;1-39.
Budson A.E., Price B.H. Memory dysfunction. N. Engl. J. Med.. 2005;352:692-699.
Derbyshire S.W., Jones A.K., Gyulai F., Clark S., Townsend D., Firestone L.L. Pain processing during three levels of noxious stimulation produces differential patterns of central activity. Pain. 1997;73:431-445.
Galpern W.R., Lang A.E. Interface between tauopathies and synucleinopathies: a tale of two proteins. Ann. Neurol.. 2006;59:449-458.
Geschwind M.D., Shu H., Haman A., Sejvar J.J., Miller B.L. Rapidly progressive dementia. Ann. Neurol.. 2008;64:97-108.
Josephs K.A. Frontotemporal dementia and related disorders: deciphering the enigma. Ann. Neurol.. 2008;64:4-14.
Marlowe W.B., Mancall E.L., Thomas J.J. Complete Kluver-Bucy syndrome in man. Cortex.. 1975;11:53-59.
Papez, 1937Papez J.W. A proposed mechanism of emotion. Arch. Neurol. Psychiatry. 38:1937;725-743.
Classic description of the mechanism of human emotion.
Passingham R.E. The Frontal Lobes and Voluntary Action. Oxford: Oxford University Press; 1993.
Penfield, Rasmussen, 1950Penfield W. Rasmussen T. The Cerebral Cortex of Man. 1950. Macmillan. New York.
Classic description of the organization of sensory and motor homunculi in the human cerebral cortex.
Peyron R., Laurent B., Garcia-Larrea L. Functional imaging of brain responses to pain: A review and meta-analysis. Neurophysiol. Clin.. 2000;30:263-288.
Sperry R.W. Lateral specialization in the surgically separated hemisphere. In: Schmidt F.O., Worden F.G., editors. The Neurosciences: Third study program. Cambridge, Mass: MIT Press; 1974:5-19.
Sperry R.W. Consciousness, personal identity and the divided brain. Neuropsychologia. 1984;17:153-166.
Victor M., Angevine J.G., Fisher C.M., Mancall E.L. Memory loss with lesions of hippocampal formation. Arch. Neurol.. 1961;5:244.