Chapter 13 Cerebellum
The cerebellum, the largest part of the hindbrain, is dorsal to the pons and medulla, and its median region is separated from them by the fourth ventricle. It is joined to the brain stem by three pairs of cerebellar peduncles, which contain afferent and efferent fibres. The cerebellum occupies the posterior cranial fossa, where it is covered by the tentorium cerebelli. It is roughly spherical but somewhat constricted in its median region and flattened; its greatest diameter is transverse. In adults, the weight ratio of cerebellum to cerebrum is approximately 1 : 10; in infants, it is approximately 1 : 20.
External Features and Relations
The cerebellum consists of two large, laterally located hemispheres that are united by a midline vermis (Figs 13.1–13.3). The superior surface of the cerebellum, which would constitute the anterior part of the unrolled cerebellar cortex, is relatively flat. The paramedian sulci are shallow, and the borders between vermis and hemispheres are indicated by kinks in the transverse fissures. The superior surface adjoins the tentorium cerebelli and projects beyond its free edge. The transverse sinus borders the cerebellum at the point where the superior and inferior surfaces meet. The inferior surface is characterized by a massive enlargement of the cerebellar hemispheres, which extends medially to overlie some of the vermis. Deep paramedian sulci demarcate the vermis from the hemispheres. Posteriorly, the hemispheres are separated by a deep vallecula, which contains the dural falx cerebelli. The inferior cerebellar surface lies against the occipital squama. The shape of the surface facing the brain stem is irregular. It forms the roof of the fourth ventricle and the lateral recesses on each side of it, while the cerebellar peduncles define the diamond shape of the ventricle when viewed from behind. Anterolaterally, the cerebellum lies against the posterior surface of the petrous part of the temporal bone.
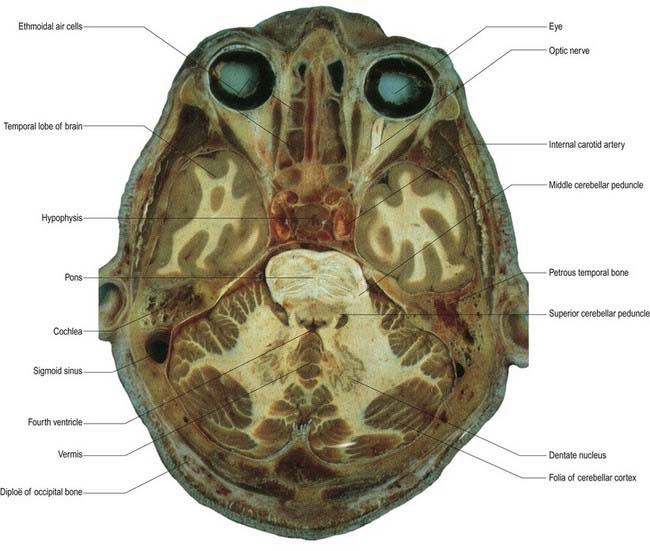
Fig. 13.1 Horizontal section through the cerebellum and brain stem.
(Courtesy of Dr. G. J. A. Maart.)
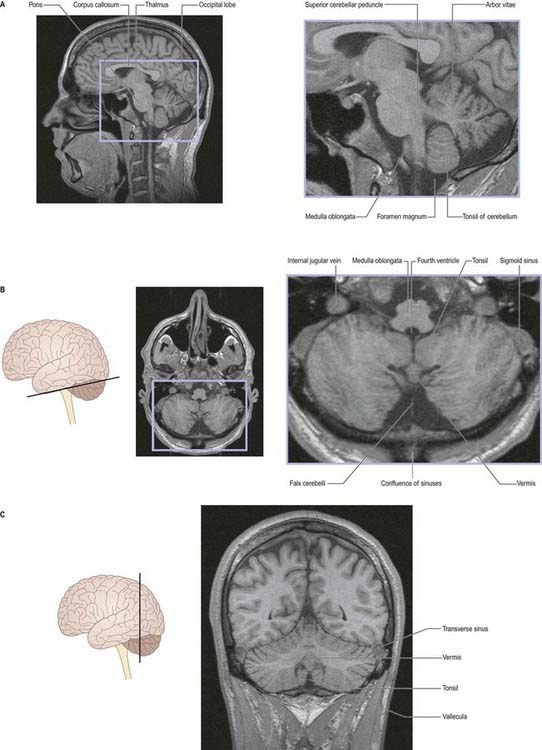
Fig. 13.2 Magnetic resonance images of the cerebellum of a 16-year-old girl. A, Sagittal slice. B, Coronal slice. C, Axial slice.
(Courtesy of Drs. J. P. Finn and T. Parrish, Northwestern University School of Medicine, Chicago, toddp@northwestern.edu.)
From the back forward, the inferior vermis is divided into the tuber, pyramis, uvula and nodule, in that order (see Fig. 13.3C). The tuber is continuous laterally with the inferior semilunar lobules and separated from the pyramis by the lunogracile fissure. The pyramis and attached biventral lobules (containing an intrabiventral fissure) are separated from the uvula and attached cerebellar tonsils by the secondary fissure. Behind the uvula, and separated from it by the median part of the posterolateral fissure, is the nodule. The tonsils are roughly spherical and overhang the foramen magnum on each side of the medulla oblongata.
Functional Divisions
The cerebellum is divided functionally into a body, with inputs mainly from the spinal cord and pontine nuclei, and a flocculonodular lobe, which has strong afferent and efferent connections with the vestibular nuclei (Fig. 13.4). The body is subdivided into a series of regions dominated by their spinal or pontine inputs. The anterior lobe, simple lobule, pyramis and biventral lobules are the main recipients of spinal and trigeminal cerebellar afferents. Pontocerebellar input dominates in the folium, tuber and uvula and in the entire hemisphere, including those regions that receive afferents from the spinal cord.
Cerebellar Peduncles
Three peduncles connect the cerebellum with the rest of the brain (Figs 13.5, 13.6). The middle cerebellar peduncle is the most lateral and by far the largest of the three. It passes obliquely from the basal pons to the cerebellum and is composed almost entirely of fibres arising from the contralateral basal pontine nuclei, with a small addition from nuclei in the pontine tegmentum. The inferior cerebellar peduncle is located medial to the middle peduncle. It consists of an outer, compact fibre tract, the restiform (Latin for ‘rope-like’) body and a medial, juxtarestiform body. The restiform body is a purely afferent system. It receives the posterior spinocerebellar tract from the spinal cord and the trigeminocerebellar, cuneocerebellar, reticulocerebellar and olivocerebellar tracts from the medulla oblongata. The juxtarestiform body is mainly an efferent system. Apart from primary afferent fibres of the vestibular nerve and secondary afferent fibres from the vestibular nuclei, it is made up almost entirely of efferent Purkinje cell axons from the vestibulocerebellum, on their way to the vestibular nuclei, and the uncrossed fibres from the fastigial nucleus. The crossed fibres from the fastigial nucleus, after passing dorsal to the superior cerebellar peduncle, enter the brain stem as the uncinate fasciculus at the border of the juxtarestiform and restiform bodies.
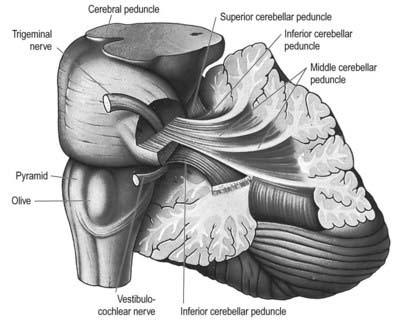
Fig. 13.5 Dissection of the left cerebellar hemisphere and its peduncles.
(Courtesy of Dr. E. B. Jamieson, University of Edinburgh.)
Internal Structure
Laterally, the medullary laminae merge into a large, central white mass that contains the four cerebellar nuclei: the dentate and the anterior (emboliform) and posterior (globose) interposed and fastigial nuclei (see Fig. 13.4). The dentate nucleus is the most lateral and largest and is an irregularly folded sheet of neurones that encloses a mass of fibres derived mainly from dentate neurones. It resembles a leather purse, the opening of which is directed medially. Fibres stream out through this so-called hilum to form the bulk of the superior cerebellar peduncle. The anterior and posterior interposed and fastigial nuclei lie medial to the dentate nucleus. The anterior interposed nucleus is continuous laterally with the dentate. The posterior interposed nucleus is medial to the anterior nucleus and is continuous with the fastigial nucleus, which is located next to the midline, bordering the fastigium (roof) of the fourth ventricle. Efferent fibres from the interposed nuclei join the superior cerebellar peduncle. A large proportion of the efferent fibres from the fastigial nucleus cross within the cerebellar white matter of the cerebellar commissure. After their decussation, they constitute the uncinate fasciculus (hook bundle), which passes dorsal to the superior cerebellar peduncle to enter the vestibular nuclei of the opposite side (see Fig. 13.6). Uncrossed fastigiobulbar fibres enter the vestibular nuclei by passing along the lateral angle of the fourth ventricle. Some fibres of the fastigial nucleus ascend in the superior cerebellar peduncle.
Cerebellar Cortex
There are three main layers: molecular, Purkinje cell and granular (Fig. 13.7). The main circuit of the cerebellum involves granule cells, Purkinje cells and neurones in the cerebellar nuclei. Granule cells receive the terminals of the mossy fibre afferents (i.e. all afferent systems except the olivocerebellar fibres). The axons of the granule cells ascend to the molecular layer, where they bifurcate into parallel fibres (so called because they are oriented parallel to the transverse fissures and perpendicular to the dendritic trees of the Purkinje cells on which they terminate). Purkinje neurones are large and are the sole output cells of the cerebellar cortex. Their axons terminate in the cerebellar nuclei and vestibular nuclei. In addition to the dense array of parallel fibres, the dendritic trees of Purkinje cells receive terminals from climbing fibres whose neurones of origin are in the inferior olivary nucleus. The cerebellar cortex thus receives two distinct types of input: olivocerebellar climbing fibres, which synapse directly on Purkinje neurones, and mossy fibres, which connect to the Purkinje cells via granular neurones whose axons are the parallel fibres.
The granular layer (see Fig. 13.7) is approximately 100 µm thick in the fissures and 400 to 500 µm thick on foliar summits. There are approximately 2.7 million granular neurones per cubic millimetre. It has been estimated that the human cerebellum contains a total of 4.6 × 1010 granule cells and that there are 3000 granule cells for each Purkinje cell.
Of the five cell types described, the first four are inhibitory, liberating γ-aminobutyric acid (GABA), and the fifth is excitatory, liberating L-glutamate. Figure 13.8 summarizes their main connections.
Purkinje cells have a specific geometry that is conserved in all vertebrate classes (see Fig. 13.7). They are arranged in a single layer between the molecular and granular layers. Individual Purkinje cells are separated by approximately 50 µm transversely and 50 to 100 µm longitudinally. Their somata measure 50 to 70 µm vertically and 30 to 35 µm transversely. The subcellular structure of the Purkinje cell is similar to that of other neurones. One distinguishing feature is subsurface cisterns, often associated with mitochondria, that are present below the plasmalemma of somata and dendrites and may penetrate into the spines. They are intracellular calcium stores, which are important links in the second messenger systems of the cell.
The connections of the cerebellum are organized in two perpendicular planes, corresponding to the planar organization of the cerebellar cortex. Efferent connections of the cortex are disposed in parasagittal sheets or bundles that connect longitudinal strips of Purkinje cells with specific cerebellar or vestibular nuclei. The climbing fibre afferents to a Purkinje cell zone from the inferior olive display a similar zonal disposition. Cerebellar output is organized in modules, with a module consisting of one or more Purkinje cell zones, their cerebellar or vestibular target nucleus and their olivocerebellar climbing fibre input. Modular function is determined by the brain stem projections of the cerebellar or vestibular target nucleus. A general feature of the modular organization of the cerebellum is that GABAergic neurones in the cerebellar nuclei project to the subnuclei of the contralateral inferior olive, which give rise to their respective climbing fibre afferents. These recurrent connections are known as nucleo-olivary pathways.
Structural and Functional Cerebellar Localization
A double, mirrored localization exists in the anterior and posterior cerebellum (Fig. 13.9). The anterior lobe, simple lobule, pyramis and adjoining lobules of the hemisphere of the posterior lobe all receive branches from the same mossy and climbing fibres and project to the same cerebellar nuclei. The efferent pathways of these regions monitor the activity in the corticospinal tract and in the subcortical motor systems descending from the vestibular nuclei and reticular formation. The inputs to the cerebellum and the outputs from it are organized according to the same somatotopic patterns, but the orientation of these patterns is reversed. The representation of the head is found principally in the simple lobule and caudally in a corresponding region of the posterior lobe. The double representation of the body follows in rough somatotopic order. Vestibular connections of the cerebellum display a similar double representation in the most rostral lobules of the anterior lobe and far caudally in the vestibulocerebellum (Fig. 13.10).
The folium, tuber, uvula, tonsil and posterior biventral lobule all receive an almost pure pontine mossy fibre input. Climbing fibres from the inferior olive and mossy fibres from the basilar and tegmental pontine nuclei relay visual and acoustic information from the respective cerebral association areas and midbrain tectum to the folium and tuber that are thought to represent a vermal visual and acoustic area (see Fig. 13.9). The efferent connections of this area travel via the fastigial nucleus to gaze centres in the pons and midbrain.
Afferent Connections of the Cerebellum
Afferent connections of the cerebellum include the mossy fibres and the climbing fibres. Mossy fibre systems terminate bilaterally in transversely oriented ‘lobular’ areas. The terminations of different mossy fibre systems overlap considerably (see Fig. 13.4). Climbing fibres from different subnuclei of the inferior olive terminate contralaterally, on discrete longitudinal strips of Purkinje cells. This longitudinal pattern closely corresponds with the zonal arrangement in the corticonuclear projection (Fig. 13.11).
Spinocerebellar and Trigeminocerebellar Fibres
The posterior spinocerebellar tract takes its origin from the posterior thoracic nucleus at the base of the dorsal horn in all thoracic segments of the spinal cord (Fig. 13.12). It enters the inferior cerebellar peduncle, gives collaterals to the cerebellar nuclei and terminates, mainly ipsilaterally, in the vermis and adjoining regions of the anterior lobe and in the pyramis and adjoining lobules of the posterior lobe. The posterior thoracic nucleus receives primary afferents of all kinds from the muscles and joints of the lower limbs, which reach the nucleus via the gracile fasciculus. It also receives collaterals from cutaneous sensory neurones. Accordingly, the tract transmits proprioceptive and exteroceptive information about the ipsilateral lower limbs. Very fast conduction is required to keep the cerebellum informed about ongoing movements. The axons in the posterior spinocerebellar tract are the largest in the central nervous system, measuring 20 µm in external diameter. The upper limb equivalent of the posterior spinocerebellar tract is the cuneocerebellar tract.
The anterior spinocerebellar tract is a composite pathway. It informs the cerebellum about the state of activity of spinal reflex arcs related to the lower limb and lower trunk. Its fibres originate in the intermediate grey matter of the lumbar and sacral segments of the spinal cord (see Fig. 13.12). They cross near their origin and ascend close to the surface as far as the lower midbrain before looping down in the superior cerebellar peduncle. Most fibres cross again in the cerebellar commissure; thus, their distributions to the cerebellar nuclei and cortex appear to be the same as those of the posterior tract.
Olivocerebellar Fibres
Localization in the Olivocerebellar System: Zones and Microzones
Climbing fibres originate exclusively from the contralateral inferior olivary complex. Projections from the different subnuclei of the inferior olive terminate as climbing fibres on longitudinal strips of Purkinje cells in the cerebellar cortex. Collaterals end on the cerebellar or vestibular target nuclei of these Purkinje cells. A longitudinal zonal arrangement is therefore characteristic of the organization of the olivocerebellar projection (see Fig. 13.11). Moreover, the olivocerebellar projection zones correspond precisely to the corticonuclear projection zones already described. Climbing fibres from the inferior olive are able to modify the cerebellar output in such a way that cells within each subnucleus of the inferior olivary complex monitor the output of a single cerebellar module.
Olivocerebellar Climbing Fibre Connections
The inferior olivary complex can be subdivided into a convoluted principal olivary nucleus and posterior and medial accessory olivary nuclei. Olivary fibres form the olivocerebellar projection to the contralateral cerebellar cortex and give off collaterals to the lateral vestibular nucleus and to the cerebellar nuclei. Climbing fibres terminate on longitudinal strips of Purkinje cells. The zonal patterns of the olivocerebellar and Purkinje–nuclear projections correspond precisely. The accessory olivary nuclei project to the vermis and the adjacent hemispheres. The caudal halves of the posterior and medial accessory nuclei innervate the vermis. The caudal part of the posterior accessory nucleus projects to Deiters’ nucleus and to the B zone of the anterior vermis. The caudal half of the medial accessory olive gives rise to a projection to the fastigial nucleus and provides climbing fibres to the A zone. The rostral halves of the accessory olives project to the pars intermedia. Climbing fibres from the rostral dorsal accessory olive give collateral projections to the emboliform nucleus and terminate in zones C1 and C3. Zone C2 receives terminals from the rostral medial accessory olive, which provides a collateral projection to the globose nucleus. The principal nucleus projects to the contralateral hemisphere (D zone), and gives collaterals to the dentate nucleus.
Vestibulocerebellar Fibres
Primary vestibulocerebellar mossy fibres are fibres of the vestibular branch of the vestibulocochlear nerve. They enter the cerebellum with the ascending branch of the vestibular nerve and pass through the superior vestibular nucleus and juxtarestiform body. They terminate, mainly ipsilaterally, in the granular layer of the nodule, caudal part of the uvula, ventral part of the anterior lobe and bottom of the deep fissures of the vermis (see Fig. 13.10A). Secondary vestibulocerebellar mossy fibres arise from the superior vestibular nucleus and the caudal portions of the medial and inferior vestibular nuclei. They terminate bilaterally not only in the same regions that receive primary vestibulocerebellar fibres but also in the flocculus, which lacks a primary vestibulocerebellar projection (see Fig. 13.10B). Some of the mossy fibres from the medial and inferior vestibular nuclei are cholinergic.
Pontocerebellar Fibres
More than 90% of fibres in the middle cerebellar peduncle belong to the corticopontocerebellar pathway. Corticopontine fibres travel in the cerebral peduncle. Fibres from the frontal lobe occupy the medial part of the peduncle, and fibres from the parietal, occipital and temporal lobes occupy the lateral part. They synapse on some 20 million neurones in corresponding regions of the basilar pons. The onward pontocerebellar mossy fibre projection is predominantly to the lateral regions of the posterior and anterior lobes, but collaterals are given off to the dentate nucleus (see Fig. 13.4A).
Efferent Connections of the Cerebellum
The output of the cerebellum consists of the inhibitory projections of the Purkinje cells to the cerebellar and vestibular nuclei, and the efferent connections of the cerebellar nuclei to motor centres in the brain stem and, through the thalamus, the motor cortex. Their effects on movement are always indirect, because there are no direct projections from the cerebellar nuclei to motor neurones. Disynaptic connections of the Purkinje cells in the anterior vermis and vestibulocerebellum with motor neurones controlling oculogyric and proximal limb muscles are mediated by the vestibular nuclei. The vermis also influences these motor neurones bilaterally through multisynaptic pathways that involve the fastigial and vestibular nuclei and the reticular formation (Fig. 13.13). The vermis cannot be considered as a single module. Each half of the vermis is composed of several modules (each made up of a longitudinal Purkinje cell zone and a target nucleus) and their supporting climbing fibre afferent projections.
Each cerebellar hemisphere influences movements of the ipsilateral extremities by way of projections to the dentate and interposed (emboliform and globose) nuclei, which in turn project to the contralateral red nucleus, thalamus and motor cortex (Fig. 13.14).
Corticonuclear and Corticovestibular Fibres
Purkinje cells of each hemivermis project to the ipsilateral fastigial and vestibular nuclei. Purkinje cells of the hemisphere project to the interposed and dentate nuclei. Although the cerebellar cortex is organized in strips of Purkinje cell zones that project to different cerebellar and vestibular nuclei, the borders between these strips are not apparent in the structure of the cortex when it is examined histologically using conventional staining methods. The vermis of the anterior lobe and simple lobule consist of two parallel strips, A and B, of Purkinje cells (see Figs 13.11, 13.13). The medial strip (A zone) projects to the rostral pole of the fastigial nucleus, and the lateral strip (B zone) projects to the lateral vestibular nucleus. The B zone does not continue beyond the simple lobule. The cortex of the entire caudal vermis, which projects to the fastigial nucleus, is included in the A zone. The folium and tuber, which represent a region of the cerebellum that receives a visual input and are involved in the accurate calibration of saccades, project to the caudal pole of the fastigial nucleus. The pyramis, uvula and nodule can be subdivided into several Purkinje cell zones. However, the significance of their connections with the cerebellar and vestibular nuclei is not well understood. Corticovestibular projections to the superior, medial and inferior vestibular nuclei, but not to the lateral nucleus, take their origin from the nodule and the adjacent region of the uvula.
The intermediate region consists of two strips of Purkinje cells (C1 and C3 zones), which project to the anterior interposed nucleus. They flank a single zone (C2) that projects to the posterior interposed nucleus (see Figs 13.11, 13.14). The rest of the hemisphere projects to the dentate nucleus. There are indications that the hemisphere can be subdivided into two zones that project to the caudolateral zone and rostromedial parts of the dentate nucleus. The neurones of the caudolateral dentate are generally smaller than those of the rostromedial dentate, and the convolutions are broader. The efferent connections of the flocculus are mainly with the superior, medial and inferior vestibular nuclei and resemble those from the nodule and uvula.
Cerebellovestibular and Cerebelloreticular Fibres
Efferent Connections of the Fastigial Nucleus
The fastigial nucleus is connected bilaterally with the vestibular nuclei and the medullary and pontine reticular formation (see Figs 13.11, 13.14). Smaller crossed connections either ascend to the midbrain and diencephalon or descend into the spinal cord. Small GABAergic neurones give rise to nucleo-olivary fibres, which terminate in the medial accessory olive. The uncinate fasciculus is the major efferent pathway of the fastigial nucleus. Its fibres cross in the rostral part of the cerebellar commissure and pass dorsal to the superior cerebellar peduncle, to enter the vestibular nuclei from their lateral side. Uncrossed fibres enter the vestibular nuclei through the juxtarestiform body (see Fig. 13.6). The distribution of the fastigial projection is bilateral, but with a contralateral preponderance (see Fig. 13.13). Crossed and uncrossed projections end in the medial and inferior vestibular nuclei. They also cross these nuclei to terminate in the medial reticular formation. Some crossed fibres can be traced caudally into the spinal cord. A small fascicle of crossed fibres from the fastigial nucleus ascends along the superior cerebellar peduncle and is distributed bilaterally to the dorsal tegmentum, central grey matter and deep layers of the superior colliculus and the nuclei of the posterior commissure. Fibres terminate bilaterally in the ventrolateral nucleus and the intralaminar nuclei of the thalamus.
Cerebellovestibular Connections
The relationship between the cerebellum and the vestibular nuclei is complex (see Fig. 13.13). In addition to the vestibulocerebellum (nodule, adjacent folia of the uvula and flocculus), the main vermis and the fastigial nucleus project to the vestibular nuclei. The vestibulocerebellum projects to the superior, medial and inferior vestibular nuclei. Neurones of these nuclei, which receive an input from the vestibular nerve and project to the nuclei controlling eye movements (vestibulo-ocular relay cells), are among the main targets of the Purkinje cells of the nodule and flocculus. Through these connections with vestibulo-ocular relay neurones, the flocculus is involved in the long-term adaptation of compensatory eye movements, the generation of smooth eye movements used to pursue an object and the suppression of the vestibulo-ocular reflex during smooth pursuit. The function of the nodule in the control of eye movement is not as well understood.
The lateral vestibular nucleus, which lacks an input from the labyrinth and receives Purkinje cell axons from the B zone of the anterior vermis, can be regarded as a displaced cerebellar nucleus. It gives rise to the lateral vestibulospinal tract, which descends to all levels of the spinal cord. It is avoided by the efferent pathways from the fastigial nucleus, which terminate more ventrally on large neurones in the magnocellular part of the medial vestibular nucleus and in the medial reticular formation. The medial and inferior vestibular nuclei receive a major input from the vestibular nerve. They give rise to bilaterally ascending and descending tracts, which course in the medial longitudinal fasciculus. The ascending tract is composed predominantly of the axons of vestibulo-ocular relay cells. The descending fibres form the medial vestibulospinal tract, which is particularly involved in head righting reflexes when the trunk is tilted.
Cerebellorubral and Cerebellothalamic Fibres
The axons of neurones in the dentate and interposed nuclei leave the cerebellum in the superior cerebellar peduncle. The superior peduncles, including their nucleo-olivary component, decussate in the caudal midbrain (see Fig. 13.14). Each peduncle then gives off a small descending branch carrying fibres that terminate in the medial reticular formation of the pons and medulla and the pontine tegmental reticular nucleus (see Fig. 13.14). The nucleo-olivary fibres join this descending branch and terminate in the inferior olive in a strictly orderly manner. The ascending branch is distributed to the midbrain and diencephalon, mainly to the red nucleus and thalamus. The anterior interposed nucleus projects to the magnocellular part of the red nucleus. In humans, this projection is very small, and it gives rise to a relatively trivial rubrospinal tract that crosses in the caudal midbrain and terminates on lateral medullary interneurones and a small number of motor neurones in the upper cervical spinal cord.
Cerebellar Functions
Motor Learning
Many motor skills require precise timing, which involves an extreme degree of cooperation between prime movers and their antagonists. For example, reading a printed page requires that the scanning eyes snap back to the beginning of a line, time after time. Even small errors may result in dyslexia, whereby slight incoordination of eye movements causes the letters of a word to appear jumbled. Clinical testing of timing can be performed easily by checking the ability to perform rhythmic movements, such as repetitive pronation–supination (Fig. 13.15).
Higher Functions
The cerebellum is currently believed to participate in higher brain functions. This is not unexpected, in view of the two-way linkages (via the thalamus) that exist between the cerebellum and the association and paralimbic areas of the cerebral cortex. Its role appears to be one of assistance rather than of generation. For example, during speech, the right posterolateral region of the cerebellum is active bilaterally, which reflects its role in coordinating the muscles involved. However, there is a right-sided predominance, which is consistent with a possible linkage (via the thalamus) with the motor speech area of the left frontal cortex. Moreover, because right lateral cerebellar activity is even greater during functional naming (e.g. ‘dig,’ ‘fly’) than during object identification (e.g. ‘shovel,’ ‘airplane’), cognitive as well as motor functions are compromised by cerebellar disorders.
Cerebellar Dysfunction
Anterior Lobe Lesions: Gait Ataxia
CASE 4 Alcoholic Cerebellar Degeneration
Discussion: The clinical features of a subacute evolving ataxia of the gait and of the legs, with good preservation of cerebellar function in the upper extremities and little if any other deficit, is typical of so-called alcoholic cerebellar degeneration occurring on a background of long-standing poor nutritional intake. The relatively restricted clinical syndrome, affecting primarily gait and the lower extremities, is explained by the observed distribution of lesions in the cerebellar cortex, involving predominantly the superior vermis and anterolateral portion of the cerebellar hemispheres—in accordance with known somatotopic localization in the cerebellar cortex (Fig. 13.16). All neurocellular components of the cerebellar cortex may be involved; Purkinje cells are most liable to damage. Secondary changes may be noted in the deep cerebellar nuclei. In some cases, features of Wernicke’s encephalopathy are noted, prompting the suggestion that both disorders reflect a vitamin, perhaps thiamine, deficiency.
Angevine J.B., Mancall E.L., Yakovlev P. The human cerebellum: a topographical atlas. Boston: Little-Brown; 1961.
Bastian A.J., Mugnaini E., Thach W.T. Cerebellum. In: Zigmond M.J., Bloom F.E., Landis S.C., Roberts J.L., Squire L.R., editors. Fundamental Neuroscience. San Diego: Academic Press; 1999:973-992.
Gebhart, Petersen, Thach, 2002Gebhart A.L. Petersen S.E. Thach W.T. Role of the posterolateral cerebellum in language. Ann. N. Y. Acad. Sci.. 978:2002;318-333.
Ivry R.B., Spencer R.M., Zelaznik H.N., Diedrichsen J. The cerebellum and event timing. Ann. N. Y. Acad. Sci.. 2002;978:302-317.
Jueptner M., Kruckenberg M. Anatomic basis of functional magnetic resonance imaging: motor system. Neuroimaging Clin. N. Am.. 2001;11:203-219.
Rae C., Haresty J.A., Dzendrowskyj T.E., et al. Cerebellar morphology in developmental dyslexia. Neuropsychologia. 2002;40:1285-1292.
Schmahmann J.D., Doyon J., Toga A.W., Petrides M., Evans A.C. MRI atlas of the human cerebellum. Orlando: Harcourt; 2000.
Schmahmann J.D., Sherman J.C. The cerebellar cognitive affective syndrome. Brain. 1998;121:561-579.
Topka H., Mescheriakov S., Boose A., et al. A cerebellar-like terminal and postural tremor induced in normal man by transcranial magnetic stimulation. Brain. 1999;122:1551-1562.
Valsamis M.P., Mancall E.L. Toxic cerebellar degeneration. Hum. Pathol.. 1973;4:513-520.
Van de Warrenburg B.P.C., Sinke R.J., Kremer B. Recent advances in hereditary spinocerebellar ataxias. J. Neuropathol. Exp. Neurol.. 2005;64:171-180.
Victor M., Adams R.D., Mancall E.L. A form of degeneration of the cerebellar cortex observed in chronic alcoholic patients. Arch. Neurol.. 1959;1:579-688.