25 Cerebellum
Introduction
The gross anatomy of the cerebellum is described briefly in Chapter 3, where it may be reviewed at this time.
Functional Anatomy
Phylogenetic and functional aspects can be combined (to an approximation) by dividing the cerebellum into strips, as shown in Figure 25.1. The median strip contains the cortex of the vermis, together with the fastigial nucleus in the white matter close to the nodule (Figure 25.2). This strip is the vestibulocerebellum; it has two-way connections with the vestibular nucleus. It controls the responses of that nucleus to signals from the vestibular labyrinth. The fastigial nucleus also projects to the gaze centers of the brainstem (Ch. 24).
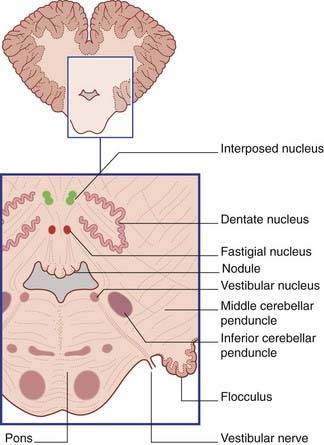
Figure 25.2 Transverse section of lower pons and cerebellum showing the position of the central and vestibular nuclei.
A paramedian strip, the spinocerebellum, includes the paravermal cortex and the globose and emboliform nuclei (Figure 25.2). The two nuclei are together called the interposed nucleus. The spinocerebellum is rich in spinocerebellar connections. It is involved in the control of posture and gait.
The remaining, lateral strip is much the largest and takes in the wrinkled dentate nucleus (Figure 25.2). This strip is the pontocerebellum, because it receives a massive input from the contralateral nuclei pontis. It is also called the neocerebellum, because the nuclei pontis convey information from large areas of the cerebral neocortex (phylogenetically the most recent). The neocerebellum is uniquely large in the human brain.
Microscopic Anatomy
The structure of the cerebellar cortex is uniform throughout. From within outward, the cortex comprises granular, piriform, and molecular layers (Figure 25.3).
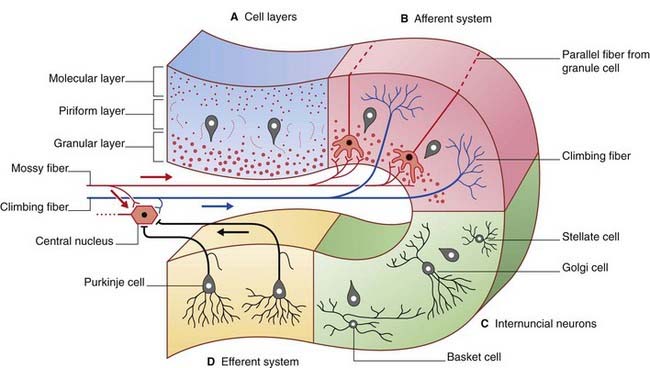
Figure 25.3 Cerebellar cortex. (A) Cell layers. (B) Afferent systems. (C) Internuncial neurons. (D) Efferent system.
The granular layer also contains Golgi cells (see later).
The final cell type in the cortex is the Golgi cell, whose dendrites are contacted by parallel fibers and whose axons divide extensively before synapsing upon the short dendrites of granule cells. The synaptic ensemble that includes a mossy fiber terminal, granule cell dendrites, and Golgi cell boutons is known as a glomerulus (Figures 25.4, 25.5).
Representation of Body Parts
Representation of body parts in the human cerebellar cortex is currently under investigation by means of fMRI. These investigations, along with some evidence from clinical cases, indicate the presence of somatotopic maps in the anterior and posterior lobes (Figure 25.7).
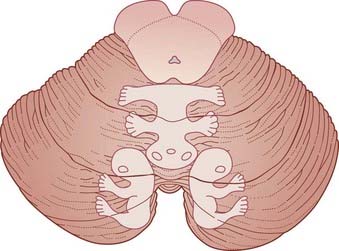
Figure 25.7 Upper surface of cerebellum showing position of somatotopic maps, based on animal experiments.
The maps have been worked out in some detail in laboratory animals during movements. The maps for movement match up with maps of skin, eye, ear, and visceral representation worked out by stimulation of body parts. The expression fractionated somatotopy refers to the patchy nature of the representation of body parts. Simple representations like those in Figure 25.7 are likely to be quite inaccurate in view of the vast areas of cortex buried in the fissures.
Figure 25.8, based on PET scans, shows simultaneous activation of the left motor cortex and right cerebellum during repetitive movements of the fingers of the right hand.
Afferent Pathways
Olivocerebellar tract
The olive receives direct ipsilateral projections from the premotor and motor areas of the cerebral cortex, and from the visual association cortex, providing an apparently suitable substrate for its activities. It is also in touch with the outside world through the spino-olivary tract (Ch. 15).
As mentioned in Chapter 15, motor adaptation is primarily a function of the cerebellum. Here the cerebellum oversees modification of routine motor programs in response to changes in the environment, e.g. walking uphill vs walking on the flat. Experimental evidence indicates that prolonged motor adaptation, e.g. walking over a period of weeks while wearing an ankle plaster cast, is accompanied by long-term potentiation (LTP) of cerebellothalamic synapses, thereby facilitating the influence of the cerebellum on the motor cortex.
Motor sequence learning, for example learning to walk during infancy, is a function of the basal ganglia (Ch. 33).
Efferent Pathways (Figure 25.10)
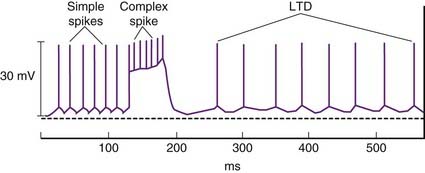
Motor learning is believed to be achieved by means of a phenomenon called long-term depression. This refers to depression of ongoing parallel fiber activity for up to several hours, following a burst of complex spikes (Figure 25.9). Both neurons concerned are glutamatergic and Purkinje dendrites possess both AMPA and metabotropic receptors. The key molecule in the interaction is the second messenger protein kinase C (PKC), which is activated by parallel fiber activity and mediates protein phosphorylation in ion channels. The molecular sequence is as illustrated in Figure 8.8. Complex spikes are associated with a large increase in intracellular calcium and this interacts with PKC to diminish the postsynaptic response of the AMPA receptors to glutamate stimulation.
Vestibulocerebellar outputs to the medial and superior vestibular nuclei control movements of the eyes through the medial longitudinal fasciculus (Chs 17, 23). A separate output to the lateral vestibular nucleus (of Deiters) of the same side controls the balancing function of the vestibulospinal tract. Some Purkinje axons skirt the fastigial nucleus and exert direct tonic inhibition on Deiters’ nucleus.
Anticipatory Function of the Cerebellum
Postural stabilization
Figure 25.11 illustrates anticipatory contraction of the gastrocnemius serving to stabilize a trunk about to receive a displacement impetus produced by contraction of the biceps brachii. In more general terms, displacement of the upper trunk away from the center of gravity by a voluntary movement of the head or upper limb is anticipated by the cerebellum. Having read instructions delivered from premotor areas of the frontal lobe (Ch. 29) concerning the intended movement, the cerebellum ensures proportionate contractions of postural muscles in a bottom-up manner, from leg to thigh to trunk, in order to keep the center of gravity in the midline between the feet. Damage to the cerebellar vermis affects normal anticipatory activation, via the lateral vestibulospinal tract, of slow-twitch, close-to-the-bone muscle bundles, with consequent failure to counter the effect of gravity displacement produced by movement of any body part (see Clinical Panel 25.1).
Damage to the anterior lobe is associated with failure of the reticulospinal tracts to anticipate the gravitational effects produced by locomotion (see Clinical Panel 25.2).
Clinical Panel 25.2 Anterior lobe lesions: gait ataxia
Instability of station with the feet together, and failure to ‘toe the line’ on walking, are present even when the eyes are open. A head tremor at 3 Hz is usually present. As the disease progresses, a peripheral sensory neuropathy may be added, giving rise to signs of sensory ataxia (Ch. 15) in addition. Tendon reflexes may be depressed in the lower limbs owing to loss of tonic stimulation of fusimotor neurons via the pontine reticulospinal tract. Consequent reduction of monosynaptic reflex activity during walking may eventually result in stretching of soft tissues, with hyperextension of the knee joint during standing.
Postural fixation
Figure 25.12 illustrates an experiment where the subject was instructed to execute sudden wrist extension and to maintain the extended wrist posture for 2 seconds, while electromyographic records were being taken from prime wrist extensors (extensors carpi radialis longus and brevis) and a prime antagonist (flexor carpi radialis). The readout revealed that the antagonist began to contract prior to completion of the movement, and that it played ‘shivering ping pong’ with the prime mover during the fixation period. The contribution of the antagonist is to prevent spontaneous oscillatory torques (tremors) caused by viscoelastic properties of the muscles. It has been shown that this ‘freeze’ arrangement can be disrupted in healthy volunteers by transcranial electromagnetic stimulation aimed at the superior cerebellar peduncle, and in disease of the lateral cerebellar lobe (see Clinical Panel 25.3).
Clinical Panel 25.3 Neocerebellar lesions: incoordination of voluntary movements
Rapid alternating movements performed under command, such as pronation/supination, become quite irregular (dysdiadochokinesia). The ‘finger-to-nose’ and ‘heel-to-knee’ tests are performed with equal clumsiness whether the eyes are open or closed – in contrast to performance in posterior column disease, where performance is adequate when the eyes are open (Ch. 15).
Clinical Disorders of the Cerebellum
Diseases involving the cerebellum usually involve more than one lobe and/or more than one of the three sagittal strips. However, characteristic clinical pictures have been described in association with lesions of the vermis (Clinical Panel 25.1), of the anterior lobe (Clinical Panel 25.2), and of the neocerebellum (Clinical Panel 25.3).
The Cerebellum and Higher Brain Functions
PET and fMRI provide information about regional changes in blood flow and oxygen consumption. ‘Movement maps’ such as those in Figure 25.8 are derived from simple repetitive movements such as opening and closing a fist. A striking feature of movement maps is how small and how medial they are. Prior to PET, it was assumed that the lateral expansion of the human posterior lobe was necessary for manual dexterity. It now appears that the lateral expansion may be associated with cognitive functions (e.g. thinking), having an anatomical base in linkages with the lateral prefrontal cortex of the cerebral hemisphere. Lateral cerebellar activity seems to be greatest during speech, with a one-sided predominance consistent with a possible linkage (via the thalamus) with the motor speech area of the dominant frontal cortex (Ch. 30). Something more than mere motor control may be involved, because lateral cerebellar activity is greater during functional naming, e.g. ‘dig’, ‘fly’, than during object identification, e.g. ‘shovel’, ‘airplane’.
Aumann T. Cerebello-thalamic synapses and motor adaptation. Cerebellum. 2002;46:69-77.
Baillieux H, De Smet HJ, Paquier Pf, De Deyn PP, Marien P. Cerebellar neurocognition: insights into the bottom of the brain. Clin Neurol Neurosurg. 2008;110:763-773.
Bastian AJ, Thach WT. Structure and function of the cerebellum. In: Manto U-B, Pandalfo M, editors. The cerebellum and its disorders. Cambridge: University Press; 2002:49-68.
Doyon J, Benali H. Reorganization and plasticity in the adult brain during learning of motor skills. Curr Opin Neurobiol. 2005;15:161-167.
FitzGerald MJT. The cerebellum. In: Standring S, et al, editors. Gray’s Anatomy. ed 39. Edinburgh: Elsevier/Churchill Livingstone; 2005:353-368.
Ito M. Cerebellar long-term depression: characterization, signal transduction, and functional roles. Phys Rev. 2001;8:1143-1195.
Middleton FA, Strick PL. Cerebellar output channels. In: Schmahmann JD, editor. The cerebellum and cognition: international review of neurobiology. San Diego: Academic Press; 1997:255-271. (vol 41)
Nitschke MF, Kleinschmidt A, Wessel K, Frahm J. Somatotopic motor representation in the human anterior cerebellum. Brain. 1996;119:1023-1029.
Schmahmann JD, Sherman JC. The cerebellar cognitive affective syndrome. Brain. 1998;121:561-579.
Topke H, Mescheriakov S, Boose A, Kunz R, Hetrich I, Seydel L, Dichgans J, Rothwell J. A cerebellar-like terminal and postural tremor induced in normal man by transcranial magnetic stimulation. Brain. 1999;125:1551-1562.
Visser JE, Carpenter MG, van der Kooij, Bloem BR. The clinical utility of posturography. Clin Neurophysiol. 2008;119:2424-2436.
Wood J, Glickstein M. The anatomy of the cerebellum. Trends Neurosci. 1998;21:370-375.