Chapter 699 Biologic Effects of Radiation on Children
Basic Principles
Radiation exposure may be natural (50%) or environmental (man-made) (50%). Radon gas accounts for the majority (37%) of natural radiation. The contribution of man-made radiation has dramatically increased to 50% from 15% in the mid-1980s. CT now is responsible for 24% of all radiation exposure and almost half of man-made radiation (see Fig. 699-1 on the Nelson Textbook of Pediatrics website at www.expertconsult.com ). Though it has been estimated that as high as 2% of all cancers in the USA may be attributable to radiation from CT studies, 75% of radiologists and emergency department physicians underestimate the radiation dose from CT. Some imaging procedures do not produce radiation (see Table 699-1 on the Nelson Textbook of Pediatrics website at www.expertconsult.com), and not all radiation-producing modalities expose a child to the same amount of radiation (see Table 699-2 on the Nelson Textbook of Pediatrics website at www.expertconsult.com
).
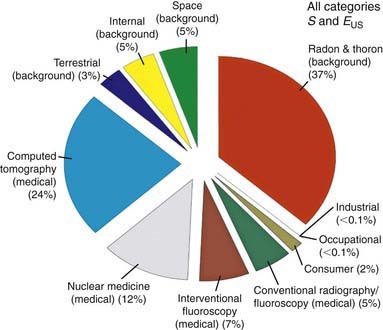
Figure 699-1 All exposure categories collective effective dose (percentage) 2006.
(From the National Council on Radiation Protection and Measurements: Medical radiation exposure of the U.S. population greatly increased since the early 1980s. www.ncrponline.org/Press_Rel/Rept_160_Press_Release.pdf. Accessed December 2, 2010.)
MODALITY | SOURCE |
---|---|
Plain film | Radiation (x-ray) |
Ultrasound | Sound beams |
Computed tomography | Radiation (x-ray) |
Magnetic resonance imaging | Magnetic field |
Nuclear medicine | Radiation (injected isotope) |
Positron emission tomography | Radiation (injected isotope) |
Table 699-2 RADIATION DOSE BY IMAGING TEST*
EXAMINATION | mrad OR mrem | SITE MEASURED |
---|---|---|
Chest—2 views | 10-20 | Entrance (skin) |
Abdominal—2 views | 50-100 | Entrance (skin) |
Fluoroscopy | ||
Nonpulsed | 300-500/min | Entrance (skin) |
Pulsed | 100-150/min | Entrance (skin) |
Computed tomography† | ||
Head | 6000 (2,000-3,000) | Mid-diameter of phantom of 16cm |
Abdomen | 3000 (1,000) | Mid-diameter of phantom of 32cm |
Nuclear medicine‡ (technetium Tc 99m mercaptoacetyltriglycine–renal) | 120 mSv | Effective dose |
Positron emission tomography‡ (brain fludeoxyglucose F 18) | 185 mSv | Effective dose, whole body |
* Background radiation = 1 mrad/day or 300 mrad/year,
† Scan explained as CT dose index (CTDI). First dose is with adult factors; second, shown in parentheses, is examination adjusted for children.
‡ Expressed as effective dose. These are rough guidelines for dose given to a 5 yr old with normal renal function.
From Valentin J: Radiation dose to patients from radiopharmaceuticals. (Addendum 2 to ICRP Publication 53.) ICRP Publication 80. Approved by the Commission in September 1997. Ann ICRP 28:1, 1998.
Nuclear medicine and positron emission tomography examinations are described by the amount of radioactivity injected (millicuries or becquerels) or are converted to effective dose (millisieverts; see later). The units of absorbed dose, as defined by the International Commission of Radiation Units, are the rad, introduced in the 1960s, and the gray (Gy), introduced in 1985. The metric used in denoting biologic response is the rem (old unit) and the sievert (Sv) (Table 699-3). Equivalent dose and effective dose are measured in Sv and mSv. Not all radiation has the same effect on biologic tissue for a given dose. Beta rays are quite superficial; alpha particles and protons cause significantly more damage than gamma radiation (x-rays) for a given absorbed dose. Diagnostic imaging uses x-rays. Each dose has a modifier, for example, skin dose, whole body dose, organ dose, or effective dose. Effective dose considers specific tissues and their radiosensitivity.
Biologic Effects of Radiation
Biologic effects of radiation are divided into two types. Deterministic effects (determined by the dose) are characterized by a threshold dose: For example, cataracts occur with an acute exposure to > 200 rad or with long-term exposure to > 500 rad (Table 699-4). Deterministic effects never occur from the doses generally used in diagnostic radiation (<0.1 Gy, 1 rad), but newer invasive procedures (therapeutic interventional) have on rare occasions led to these effects. Stochastic (random) effects are of greater concern because they can occur at any dose; that is, there is no threshold, with the probability of an effect increasing with rising dose. These effects can be caused by any radiation striking vulnerable tissue (most importantly DNA, but cytoplasm also may be at risk) and causing irreversible damage. These effects lead to the linear nondose threshold (LNT) concept, which states that radiation damage increases with rising dose in a linear fashion. This concept stresses that no level of radiation exposure can be considered to be absolutely safe.
INJURY | APPROXIMATE THRESHOLD |
---|---|
SKIN | |
Transient erythema | 200 rad (2 Gy) |
Dry desquamation | 1,000 rad (10 Gy) |
Moist desquamation | 1,500 rad (15 Gy) |
Temporary epilation | 200 rad (2 Gy) |
Permanent epilation | 700 rad (7 Gy) |
EYES | |
Cataracts (acute) | >200 rad (2.0 Gy) |
Modified from Hall EJ: Radiobiology for the radiologist, ed 5, Philadelphia, 2000, Lippincott Williams & Wilkins.
When DNA damage occurs, aberrations are produced in chromosomes, resulting in an unstable aberration (usually lethal to dividing cells) or stable aberration. Stable aberrations can result in failure of chromosomes to reunite (leading to deletions) or in abnormal rearrangement of chromosomes, such as reciprocal translocation or aneuploidy. Although it is logical to think that these abnormalities in chromosomes lead to mutations that can activate oncogenes or protooncogenes or cause mutations in tumor-suppressor genes (Chapter 486), few radiation-induced cancers show specific translocations such as would be associated with activation of specific oncogenes or known tumor-suppressor genes. An exception is the radiation induction of papillary thyroid carcinoma in children, which probably results from activation of the RET oncogene (Chapter 500).
Radiation carcinogenesis seems to be a progressive multistep process composed of three independent stages: morphologic changes, cellular immortality, and tumorigenicity. Radiation exposure induces cellular genomic instability. This instability is transmitted to a cell’s progeny, resulting in a continued elevation in the rate at which genetic changes arise in the subsequent generations of the irradiated cell (Fig. 699-2).
A longitudinal study of the lifetime risks of excess cancer mortality secondary to irradiation has been evaluated in atomic bomb survivors. More than 86,000 survivors have been followed for more than 50 yr since exposure. Individual radiation doses were estimated by considering the person’s location in relation to distance from the epicenter and individual shielding situations. Most of the exposure was direct gamma irradiation, with some neutron exposure. Age at exposure influences sensitivity to radiation-induced cancers (Fig. 699-3). Compared with the middle-aged adult, children are 10 times more sensitive to radiation-induced carcinogenesis, and the youngest neonate is more sensitive than the older child. Because of the higher risks associated with breast and thyroid cancer, girls are more sensitive than boys. It must be understood that cancer rates in this study are mortality figures. The incidence of cancer in this population is estimated to be two times greater than mortality.
The doses used in diagnostic radiology for multislice CT scans overlap with low-dose induced cancer in atomic bomb survivors (Fig. 699-4). It has been estimated that the lifetime risk of cancer following head and abdominal CT scans in children is 1 : 1000. Therefore, since stochastic effects are random but increase with rising dose, it is mandatory that we use the lowest dose necessary to get diagnostic images. The advent of digital picture archiving communication systems (PACSs) utilizes post-processing algorithms and can make all the pictures good. This obviates the imager’s ability to know whether enough or too much radiation was given. It does not allow the imager to determine whether the patient received “as low as reasonably achievable” (ALARA) radiation dosing. It is for this reason that some radiation metric should appear on each image.
Most childhood tumors occur sporadically, but 10-15% of cases have a strong familial association. Familial tumors have specific chromosomal deletions in common. In some of these tumors (retinoblastoma), the two-hit hypothesis by Knudson is apparent (Chapter 485). It is not coincidental that individuals with many of the congenital diseases are at risk for the development of tumors after irradiation. Diseases that are associated with sensitivity to radiation are listed in Table 699-5.
Table 699-5 INHERITED HUMAN SYNDROMES ASSOCIATED WITH SENSITIVITIES TO X-RAYS
Modified from Slovis TL, Frush DP, Berdon WE, et al: Biologic effects of diagnostic radiation on children. In Slovis TL, editor: Caffey’s pediatric diagnostic imaging, ed 11, Philadelphia, 2008, Mosby, p 5; and Hall EJ: Radiobiology for the radiologist, ed 6, Philadelphia, 2006, Lippincott Williams & Wilkins, p 41.
Decreasing Unnecessary Diagnostic Radiation in Children While Still Obtaining Diagnostic Images—Our Responsibility
Reducing Radiation from the CT Examination
CT is one of our most valuable diagnostic tools, and we must find ways to use it with a high benefit-to-risk ratio. The problem is a small individual risk but a large public health issue. The Society for Pediatric Radiology has joined with the American Academy of Pediatrics and a large number of medical organizations to form the ImageGently campaign. Its website, www.imagegently.org, provides up-to-date information on radiation safety.
Radiation Therapy—Acute and Late Effects
The late effects of therapy (beginning > 3 months after therapy) are numerous (Table 699-6).
Table 699-6 LATE EFFECTS OF RADIATION THERAPY IN CHILDREN TREATED FOR CANCER
SYSTEM | LATE EFFECT | DOSE (Gy) |
---|---|---|
Musculoskeletal | Muscular hypoplasia | >20 |
Scoliosis, kyphosis, lordosis | 10-20 | |
Osteocartilaginous exostosis | ? | |
Neuroendocrine (cranial or cranial spinal) | Impaired growth hormone | >18 |
Adrenocorticotropic hormone deficiency | >40 | |
Thyrotropin-releasing deficiency | >40 | |
Precocious puberty (females mostly) | >20 | |
Gonadotropin deficiency | <40 | |
Gonad failure | Ovarian failure | 4-12 |
Testicular failure | >3 | |
Central nervous system dysfunction* | Structured changes | >18 |
Cognitive changes | ? | |
Other | Pulmonary fibrosis | |
Nephropathy | ||
Liver failure | ||
Arteritis | ||
Eye impairment | ||
Ear impairment | ||
Bone marrow dysfunction | ||
Cardiac impairment |
* With intrathecal chemotherapy (methotrexate).
Derived from Halperin EC, Constine LS, Tarbell NJ, et al, editors: Pediatric radiation oncology, ed 3, Philadelphia, 1999, Lippincott Williams & Wilkins.
Second cancers account for 6-10% of all cancers in children or adults. Among children in the Childhood Cancer Survivor Study, there is a cumulative incidence of second neoplasms of 3.2% at 20 years from original diagnosis. Primary malignancies with the highest cumulative incidence of a second neoplasm in the order of frequency are: Hodgkin’s disease (7.6), soft-tissue sarcoma (4.0), bone (3.3), leukemia (2.1), central nervous system (CNS) (2.1), and non-Hodgkin (1.9). This reflects an overall standard incidence rate (SIR) of 6.38% (Fig. 699-5). The most prevalent second tumors are bone, breast, thyroid, and CNS (Fig. 699-6).
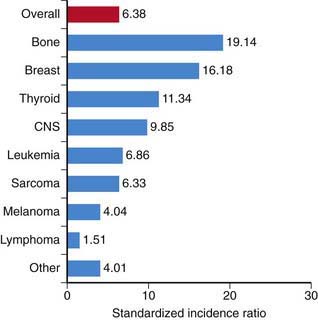
Figure 699-6 Standardized incidence ratio by type of second malignancy. CNS, central nervous system.
(Robison LL: Treatment-associated subsequent neoplasms among long-term survivors of childhood cancer: the experience of the Childhood Cancer Survivor Study, Pediatr Radiol 39[Suppl 1]:S32–S37, 2009.)
Table 699-7 relates second cancers to primary cancer and latency period. Almost 70% of the second neoplasms are in the field of the original irradiation. Radiation therapy increases the risk of second cancers in a dose-dependent manner for nongenetic neoplasms.
Irradiation also has other effects specific to children (see Table 699-6). Scoliosis and hypoplasia of bones may occur if fractionated treatment schemes exceed 4,000 rad. Fractionated doses higher than 2,500 rad can result in slipped capital femoral epiphyses. An increase in the incidence of benign osteochondromas also has been reported after childhood irradiation. Chest wall irradiation of girls (besides causing breast cancer) may impair breast development and/or cause fibrosis and atrophy of breast tissue.
Whole-Body Irradiation
Uncontrolled Large- or Small-Scale Exposure to Radiation
Large-scale exposure to radiation can occur in an event of nuclear accidents, war, or terrorist attacks (Chapters 36 and 704). Radiation as well as explosive and thermal injury need to be considered.
Clinical Manifestations
Table 699-8 presents dose-effect relationships for acute whole-body penetrating radiation. A large single exposure of penetrating radiation can result in acute radiation syndrome. The signs and symptoms of this syndrome result from damage to major organ systems that have different levels of radiation sensitivity, modulated by the rate at which the radiation exposure occurred. Delivery of 100 rads in 1 min would be symptomatic, but delivery 1 rad/day for 100 days would not be symptomatic.
Table 699-8 DOSE-EFFECT RELATIONSHIPS AFTER ACUTE WHOLE-BODY IRRADIATION FROM GAMMA RAYS OR X-RAYS
WHOLE-BODY ABSORBED DOSE, RAD (Gy) | FINDINGS |
---|---|
5 (0.05) | Asymptomatic |
15 (0.15) | Asymptomatic (but chromosome aberrations may be present in cultured peripheral lymphocytes) |
50 (0.5) | Asymptomatic (minor depression of white blood cell and platelet counts in a few persons) |
100 (1.0) | Nausea and vomiting in approximately 10% of patients within 2 days of exposure |
200 (2.0) | Nausea and vomiting in most persons exposed, with clear hematologic depression |
400 (4.0) | Nausea, vomiting, and diarrhea within 48 hr; 50% mortality without medical treatment |
600 (6.0) | 100% mortality within 30 days from bone marrow failure without medical treatment |
5,000 (50.0) | Cardiovascular collapse and central nervous system damage, with death in 24-72 hr |
The hematopoietic syndrome results from acute whole-body doses above 200 rad. A prodromal phase consists of nausea and vomiting within the first 12 hr, with symptoms usually lasting up to 48 hr. A latent period of 2-3 wk, during which patients may feel quite well, follows. Although patients are asymptomatic, bone marrow impairment has occurred. The most obvious laboratory finding is lymphocyte depression (Table 699-9). Maximal bone marrow depression occurs approximately 30 days after exposure, when hemorrhage and infection can be major problems. If the bone marrow was not completely eradicated, a recovery phase then ensues. This radiation effect is similar to what occurs when whole-body irradiation (given as 1200 rads in 2 treatments) is used to obliterate the bone marrow in children with leukemia before bone marrow transplantation.
Table 699-9 EXPECTED OUTCOME BASED ON ABSOLUTE LYMPHOCYTE COUNT AFTER ACUTE PENETRATING WHOLE-BODY IRRADIATION
MINIMAL LYMPHOCYTE COUNT WITHIN FIRST 48 HR AFTER EXPOSURE | PROGNOSIS |
---|---|
1,000-3,000 (normal range) | No significant injury |
1,000-1,500 | Significant but probably nonlethal injury, good prognosis |
500-1,000 | Severe injury, fair prognosis |
100-500 | Very severe injury, poor prognosis |
<100 | Lethal without compatible bone marrow donor |
Localized Irradiation
Clinical Manifestations
The skin changes that occur after a single acute, localized irradiation are listed in Table 699-10.
Table 699-10 SKIN CHANGES AFTER A SINGLE, ACUTE, LOCALIZED RADIATION EXPOSURE*
ABSORBED DOSE (Gy) | CHANGE |
---|---|
3-4 | Epilation in 2-3 wk |
10-15 | Threshold for erythema; appears 18-20 days after exposure at lower doses; may appear within a few hours at higher doses |
20 | Moist desquamation, possible ulceration |
25 | Ulceration with slow healing |
30-50 | Blistering, necrosis at 3 wk |
100 | Blistering, necrosis at 1-2 wk |
* Data from Gusev I, Guskova AK, Mettler FA Jr editors: Medical management of radiation accidents, ed 2, Boca Raton, FL, 2001, CRC Press.
Treatment
Skin therapy is directed at prevention of infections. Treatment of localized injuries usually involves plastic surgery and grafting, if the radiation exposure was not very penetrating (Chapter 68). The nature of the surgery depends on the dose at various depths in tissue and the location of the lesion. The full expression of radiation injury often is not apparent for 1-2 yr, owing to slow arteriolar narrowing that can cause delayed necrosis. After relatively penetrating radiation, amputation may be necessary because of obliterative changes in small vessels.
Internal Contamination
Treatment
The most effective treatment requires knowledge of both the radionuclide and the chemical form. Treatment must be instituted quickly to be effective (Table 699-11). Removal treatment involves cleaning a contaminated wound and performing stomach lavage or administration of cathartics in the case of ingestion. Administration of alginate-containing antacids (e.g., Gaviscon) also usually helps in removal by decreasing absorption in the gastrointestinal tract. An example of blocking therapy is the administration of potassium iodine or other stable iodine-containing compounds to patients with known internal contamination with radioactive iodine. The stable iodine effectively blocks the thyroid, although its effectiveness decreases rapidly as time elapses after the contamination. The recommended dose of potassium iodine is 16 mg for neonates; 32 mg for children ≤ age 3 yr; and 65 mg for children age 3-18 yr. Each dose protects for only 1 day. Dilution therapy is used in cases of tritium (radioactive hydrogen as water) contamination. Forcing fluids promotes excretion. Cases of internal contamination with transuranic elements (americium and plutonium) may require chelation therapy with calcium diethylene triamine pentaacetic acid (DTPA).
Table 699-11 SPECIFIC THERAPY FOR INTERNAL RADIATION CONTAMINATION
RADIONUCLIDE | THERAPEUTIC APPROACH |
---|---|
Tritium | Dilution (force fluids) |
Iodine 125 or iodine 131 | Blockage (SSKI [saturated solution of potassium iodide] or potassium iodide), mobilization (antithyroid drugs) |
Cesium 134 or cesium 137 | Reduction of gastrointestinal absorption (Prussian blue) |
Strontium 89 or strontium 90 | Reduction of absorption (aluminum phosphate gel antacids), blockage (strontium lactate), displacement (oral phosphate), mobilization (ammonium chloride or parathyroid extract) |
Plutonium and other transuranic elements | Chelation with zinc or calcium diethylenetriamine pentaacetic acid (investigational agents) |
Unknown | Reduction of absorption (emetics, lavage, charcoal, or laxatives) in cases of ingestion |
From Mettler FA, Voelz GL: Major radiation exposure—what to expect and how to respond. N Engl J Med 346:1554, 2002.
External Contamination
The presence of external radioactive contamination on a patient’s skin is not an immediate medical emergency. Management involves removing and controlling the spread of radioactive materials. If a patient has suspected surface contamination and no physical injuries, decontamination can be performed relatively easily. If substantial physical trauma or other life-threatening injuries are combined with external contamination, surface decontamination should proceed only after the patient has been stabilized physiologically. In many accident situations, essential medical care is delayed inappropriately by hospital emergency staff because of fear of radiation or spread of contamination in the hospital. After a radiation accident, triaging of patients is critical and is based on exposure and symptoms (Fig. 699-7).
American College of Radiology. Practice guidelines and technical standards. Reston, VA: American College of Radiology; 2003.
Becker SM. Protecting public health after radiation emergencies. BMJ. 2011;342:d1968.
Bernal B, Altman NR. Evidence-based medicine: neuroimaging of seizures. Neuroimaging Clin North Am. 2003;13:211-224.
Berrington de Gonzalez A, Darby S. Risk of cancer from diagnostic x-rays: estimates for the UK and 14 other countries. Lancet. 2004;363:345-351.
Berrington de Gonzalez A, Mahesh M, Kim KP, et al. Projected cancer risks from computed tomographic scans performed in the United States in 2007. Arch Intern Med. 2009;169:2071-2077.
Bhatia S, Sklar S. Second cancers in survivors of childhood cancer. Nat Rev Cancer. 2002;2:124-132.
Brenner DJ, Elliston CD, Hall EJ, et al. Estimated risks of radiation-induced fatal cancer from pediatric CT. AJR Am J Roentgenol. 2001;176:289-296.
Brenner DJ, Hall EJ. Computed tomography—an increasing source of radiation exposure. N Engl J Med. 2007;357:2277-2284.
Brenner DJ, Hricak H. Radiation exposure from medical imaging. JAMA. 2010;304:208-209.
Chhem RK, Meghzifene A, Czarwinski R. Towards better and safer use of radiation in medicine. Lancet. 2010;375:1328-1330.
Cuthbertson DJ, Davidson J. What to tell patients about radioiodine therapy. BMJ. 2006;333:271-272.
Doll R, Wakeford R. Risk of childhood cancer from fetal irradiation. Br J Radiol. 1997;70:130-139.
Fazel R, Krumholz HM, Wang Y, et al. Exposure to low-dose ionizing radiation from medical imaging procedures. N Engl J Med. 2009;36:849-857.
Frush DP, Donnelly LF, Rosen NS. Computed tomography and radiation risks: what pediatric health care providers should know. Pediatrics. 2003;112:951-957.
Gangopadhyay KK, Sundram F, De P. Triggering radiation alarms after radioiodine treatment. BMJ. 2006;333:293-294.
Gusev I, Guskova AK, Mettler FAJr, editors. Medical management of radiation accidents, ed 2, Boca Raton, FL: CRC Press, 2001.
Hall EJ. Radiation biology for pediatric radiologists. Pediatr Radiol. 2009;39(Suppl 1):S57-S64.
Huda W, Atherton JV, Ware DE, et al. An approach for the estimation of effective radiation dose at CT in pediatric patients. Radiology. 1997;203:417-422.
Huda W. Effective dose to adult and pediatric patients. Pediatr Radiol. 2002;32:272-279.
Imaizumi M, Usa T, Tominaga T, et al. Radiation dose-response relationships for thyroid nodules and autoimmune thyroid diseases in Hiroshima and Nagasaki atomic bomb survivors 55–58 years after radiation exposure. JAMA. 2006;295:1011-1022.
Jaffurs D, Denny A. Diagnostic pediatric computed tomographic scans of the head: actual dosage versus estimated risk. Plast Reconstr Surg. 2009;124:1254-1260.
Kal HB, Struikmans H. Radiotherapy during pregnancy: fact and fiction. Lancet Oncol. 2005;6:328-333.
Karachaliou F, Simatos G, Batika P, et al. Endocrine consequences of childhood malignancies. J BUON. 2009;14:27-32.
Kasatkinan EP, Shilin DE, Rosenbloom AL, et al. Effects of low level radiation from the Chernobyl accident in a population with iodine deficiency. Eur J Pediatr. 1997;156:916.
Kleinerman RA. Radiation-sensitive genetically susceptible pediatric sub-populations. Pediatr Radiol. 2009;39(Suppl 1):S27-S31.
Koenig TR, Wolff D, Mettler FA, et al. Skin injuries from fluoroscopically guided procedures: Part I: characteristics of radiation injury. AJR Am J Roentgenol. 2001;177:3-11.
Linet MS, Kim KP, Rajaraman P. Children’s exposure to diagnostic medical radiation and cancer risk: epidemiologic and dosimetric considerations. Pediatr Radiol. 2009;39(Suppl 1):S4-S26.
Martin D, Semelka RC. Health effects of ionizing radiation from diagnostic CT. Lancet. 2006;367:1712-1714.
McLean D. Computed tomography doses in children. Lancet. 2004;363:1178.
Medical The. Letter: Potassium iodide for thyroid protection in a nuclear-accident. Med Lett. 2011;53:25-26.
Mettler FAJr. Medical resources and requirements for responding to radiological terrorism. Health Phys. 2005;89:488-493.
Mettler FA, Voelz GL. Major radiation exposure—what to expect and how to respond. N Engl J Med. 2002;346:1554-1561.
National Council on Radiation Protection and Measurements http://NCRPonline.org. Accessed December 2, 2010
Oeffinger KC, Mertens AC, Sklar CA, et al. Chronic health conditions in adult survivors of childhood cancer. N Engl J Med. 2006;355:1572-1582.
Pierce DA, Preston DL. Radiation-related cancer risks at low doses among atomic bomb survivors. Radiat Res. 2000;154:178-186.
Pierce DA, Shimizu Y, Preston DL, et al. Studies of the mortality of atomic bomb survivors. Report 12, part 1. Cancer: 1950–1990. Radiat Res. 1996;146:1-27.
Preston DL, Ron E, Yonehara S, et al. Tumors of the nervous system and pituitary gland associated with atomic bomb radiation exposure. J Natl Cancer Inst. 2002;94:1555-1563.
Puch-Kapst K, Juran R, Phys D, et al. Radiation exposure in 212 very low and extremely low birth weight infants. Pediatrics. 2009;124:1556-1564.
Robison LL. Treatment-associated subsequent neoplasms among long-term survivors of childhood cancer: the experience of the Childhood Cancer Survivor Study. Pediatr Radiol. 2009;39(Suppl 1):S32-S37.
Salamipour H, Jimenez RM, Bree SL, et al. Multidetector row CT in pediatric musculoskeletal imaging. Pediatr Radiol. 2005;35:555-564.
Shimizu Y, Kodama K, Nishi N, et al. Radiation exposure and circulatory disease risk: Hiroshima and Nagasaki atomic bomb survivor data, 1950–2003. BMJ. 2010;340:b5349.
Slovis TL. Children, computed tomography radiation dose, and the as low as reasonably achievable (ALARA) concept. Pediatrics. 2003;112:971-972.
Smith-Bindman R, Lipson J, Marcus R, et al. Radiation dose associated with common computed tomography examinations and the associated lifetime attributable risk of cancer. Arch Intern Med. 2009;169:2078-2086.
Turai I, Veress K, Günalp B, et al. Medical response to radiation incidents and radionuclear threats. BMJ. 2004;328:568-572.