CHAPTER 1 Basic structure and function of cells
CELL STRUCTURE
GENERAL CHARACTERISTICS OF CELLS
The shapes of mammalian cells vary widely depending on their interactions with each other, their extracellular environment and internal structures. Their surfaces are often highly folded when absorptive or transport functions take place across their boundaries. Cell size is limited by rates of diffusion, either that of material entering or leaving cells, or of diffusion within them. Movement of macromolecules can be much accelerated and also directed by processes of active transport across membranes and by transport mechanisms within the cell. According to the location of absorptive or transport functions, apical microvilli (Fig. 1.1) or basolateral infoldings create a large surface area for transport or diffusion.
CELLULAR ORGANIZATION
Each cell is contained within its limiting plasma, or surface, membrane which encloses the cytoplasm. All cells except mature red blood cells also contain a nucleus that is surrounded by a nuclear membrane or envelope (Fig. 1.1, Fig. 1.2). The nucleus includes the genome of the cell contained within the chromosomes, the nucleolus, and other sub-nuclear organelles. The cytoplasm contains several systems of organelles. These include a series of membrane-bound structures that form separate compartments within the cytoplasm, such as rough and smooth endoplasmic reticulum, Golgi apparatus, lysosomes, peroxisomes, mitochondria and vesicles for transport, secretion and storage of cellular components. There are also structures that lie free in the non-membranous, cytosolic compartment. They include ribosomes and several filamentous protein networks known collectively as the cytoskeleton. The cytoskeleton determines general cell shape and supports specialized extensions of the cell surface (microvilli, cilia, flagella). It is involved in the assembly of new filamentous organelles (e.g. centrioles) and controls internal movements of the cytoplasm and cytoplasmic vesicles. The cytosol contains many soluble proteins, ions and metabolites.
Cell polarity and domains
Epithelia (including endothelia and mesothelia) are organized into sheets or more complex structures (see Ch. 2) with very different environments on either side. These cells actively transfer macromolecules and ions between the two surfaces and are thus polarized in structure and function (Fig. 1.3). In polarized cells, particularly in epithelia, the cell is generally subdivided into domains that reflect the polarization of activities within it. The free surface, e.g. that facing the intestinal lumen or airway, is the apical surface, and its adjacent cytoplasm is the apical cell domain. This is where the cell interfaces with a specific body compartment (or, in the case of the epidermis, with the outside world). The apical surface is specialized to act as a barrier, restricting access of substances from this compartment to the rest of the body. Specific components are selectively absorbed from, or added to, the external compartment by the active processes, respectively, of active transport and endocytosis inwardly or exocytosis and secretion outwardly. The apical surface is often covered with small protrusions of the cell surface, microvilli, which increase the surface area, particularly for absorption.
PLASMA MEMBRANE
Combinations of biochemical, biophysical and biological techniques have revealed that lipids are not homogenously distributed in membranes, but that some are organized into microdomains in the bilayer, called ‘detergent-resistant membranes’ or lipid ‘rafts’, rich in sphingomyelin and cholesterol (Morris et al 2004). The ability of select subsets of proteins to partition into different lipid microdomains has profound effects on their function, e.g. in T-cell receptor and neurotrophin signalling. The highly organized environment of the domains provides a signalling, trafficking and membrane fusion environment very different from that found in the disorganized fluid mosaic membrane.
In the electron microscope, membranes fixed and contrasted by heavy metals such as osmium appear in section as two densely stained layers separated by an electron-translucent zone – the classic unit membrane (Fig. 1.4). The total thickness is about 5 nm. Freeze-fracture cleavage planes usually pass along the midline of each membrane, where the hydrophobic tails of phospholipids meet. This technique has also demonstrated intramembranous particles embedded in the lipid bilayer; these are in the 5–15 nm range and in most cases represent large transmembrane protein molecules or complexes of molecules. Intramembranous particles are distributed asymmetrically between the two half-membranes, usually adhering more to one face than to the other. In plasma membranes, the inner or protoplasmic (cytoplasmic) half-membrane carries most particles, seen on its surface facing the exterior (the P face). Where they have been identified, particles usually represent channels for the transmembrane passage of ions or molecules.
The cell coat (glycocalyx)
The plasma membrane differs structurally from internal membranes in that it possesses an external, diffuse, carbohydrate-rich coat, the cell coat or glycocalyx. The cell coat forms an integral part of the plasma membrane, projecting as a diffusely filamentous layer 2–20 nm or more from the lipoprotein surface (see Fig. 1.5). The overall thickness of the plasma membrane is therefore variable, but is typically 8–10 nm. The cell coat is composed of the carbohydrate portions of glycoproteins and glycolipids embedded in the plasma membrane (Fig. 1.4).
Cell surface contacts
The plasma membrane is the surface which establishes contact with other cells and with structural components of extracellular matrices. These contacts may have a predominantly adhesive role, or initiate instructive signals within and between cells, or both; they frequently affect the behaviour of cells. Structurally, there are two main classes of contact, both associated with cell adhesion molecules. One class is associated with specializations at discrete regions of the cell surface that are ultrastructurally distinct. These are described on page 6. The second, general, class of adhesive contact has no obvious associated ultrastructural features.
General adhesive contacts
Calcium-dependent adhesion molecules
Cadherins, selectins and integrins are calcium-dependent adhesion molecules. Cadherins are transmembrane proteins, with five heavily glycosylated external domains. They are responsible for strong general intercellular adhesion, as well as being components of some specialized adhesive contacts, and are attached by linker proteins (catenins) at their cytoplasmic ends to underlying cytoskeletal fibres (either actin or intermediate filaments). Different cell types possess different members of the cadherin family, e.g. N-cadherins in nervous tissue, E-cadherins in epithelia, and P-cadherins in the placenta. These molecules bind to those of the same type in other cells (homophilic binding), so that cells of the same class adhere to each other preferentially, forming tissue aggregates or layers, as in epithelia. For a review of cadherin-mediated adhesion in morphogenesis, see Gumbiner (2005).
Selectins are found on leukocytes, platelets and vascular endothelial cells. They are transmembrane lectin glycoproteins that can bind with low affinity to the carbohydrate groups on other cell surfaces to permit movement between the two, e.g. the rolling adhesion of leukocytes on the walls of blood vessels (p. 136). They function cooperatively in sequence with integrins, which strengthen the selectin adhesion.
Calcium-independent adhesion molecules
The best known calcium-independent adhesion molecules are glycoproteins that have external domains related to immunoglobulin molecules. Most are transmembrane proteins. Some are entirely external, either attached to the plasma membrane by a glycosylphosphatidylinositol anchor, or secreted as soluble components of the extracellular matrix. Different types are expressed in different tissues. Neural cell adhesion molecules (N-CAMs) are found on a number of cell types, but are expressed widely by neural cells. Intercellular adhesion molecules (I-CAMs) are expressed on vascular endothelial cells. Cell adhesion molecule binding is predominantly homophilic, although some use a heterophilic mechanism, e.g. vascular intercellular adhesion molecule (VCAM), which can bind to integrins.
For further information on all aspects of cell adhesion molecules and intercellular contacts, see Pollard & Earnshaw (2007).
Specialized adhesive contacts
Specialized adhesive contacts, some of which mediate activities other than simple mechanical cohesion, are localized regions of the cell surface with particular ultrastructural characteristics. Three major classes exist: occluding, adhesive and communicating junctions (Fig. 1.5).
Occluding junctions (tight junctions, zonula occludens)
Occluding junctions create diffusion barriers in continuous layers of cells, including epithelia, mesothelia and endothelia, and prevent the passage of materials across the cellular layer through intercellular spaces. They form a continuous belt (zonula) around the cell perimeter, near the apical surface in cuboidal or columnar epithelial cells. At a tight junction, the membranes of the adjacent cells come into contact, so that the gap between them is obliterated. Freeze-fracture electron microscopy shows that the contacts between the membranes lie along branching and anastomosing ridges formed by the incorporation of chains of intramembranous protein particles on the P face of the lipid bilayer (Fig. 1.5C).
This arrangement ensures that substances can only pass through the layer of cells by diffusion or transport through their apical membranes and cytoplasm. The cells thus selectively modify the environment on either side of the layer. Occluding junctions also create regional differences in the plasma membranes of the cells in which they are found. For example, in epithelia, the composition of the apical plasma membrane differs from that of the basolateral regions (see Fig. 1.3), and this allows these regions to engage in functions such as directional transport of ions and uptake of macromolecules. Because tight junctions have high concentrations of fixed transmembrane proteins, they act as barriers to lateral diffusion of lipid and protein within membranes. The integrity of tight junctions is calcium-dependent. Cells can transiently alter the permeability of their tight junctions to increase passive paracellular transport in some circumstances.
Adhesive junctions
Desmosomes (maculae adherentes)
Desmosomes are limited, plaque-like areas of particularly strong intercellular contact. They can be located anywhere on the cell surface. In epithelial cells, there may be a circumferential row of desmosomes parallel to the tight and intermediate junctional zones, an arrangement that forms the third, most basally situated, component of the epithelial apical junctional complex (Fig. 1.5). The intercellular gap is approximately 25 nm, is filled with electron-dense filamentous material running transversely across it and is also marked by a series of densely staining bands running parallel to the cell surfaces. Adhesion is mediated by calcium-dependent cadherins, desmoglein and desmocollin. Within the cells on either side, a cytoplasmic density underlies the plasma membrane and includes the anchor proteins desmoplakin and plakoglobin, into which the ends of intermediate filaments are inserted. The type of intermediate filament depends on cell type, e.g. keratins are found in epithelia and desmin filaments in cardiac muscle cells. Desmosomes form strong anchorage points, likened to spot-welds, between cells subject to mechanical stress, e.g. in the prickle cell layer of the epidermis, where they are extremely numerous and large.
Hemidesmosomes
Hemidesmosomes are best known as anchoring junctions between the bases of epithelial cells and the basal lamina. Ultrastructurally, they resemble a single-sided desmosome, anchored on one side to the plasma membrane, and on the other to the basal lamina and adjacent collagen fibrils (Fig. 1.5D and see Fig. 7.5). On the cytoplasmic side of the membrane there is a dense plaque into which keratin filaments are inserted. Hemidesmosomes use integrins as their adhesion molecules, whereas desmosomes use cadherins.
Gap junctions (communicating junctions)
Gap junctions resemble tight junctions in transverse section, but the two apposed lipid bilayers are separated by an apparent gap of 3 nm which is bridged by numerous transmembrane channels (connexons). Connexons are formed by a ring of six connexin proteins in each membrane. Their external surfaces meet those of the adjacent cell in the middle. A minute central pore links one cell to the next (Fig. 1.5). These channels may exist in small numbers, and this makes them difficult to detect structurally. However, they lower the transcellular electrical resistance and so can be detected by microelectrodes. Larger assemblies of many thousands of channels are often packed in hexagonal arrays (Fig. 1.5B). Such junctions form limited attachment plaques rather than continuous zones, and so allow free passage of substances within the adjacent intercellular space, unlike tight junctions. They occur in numerous tissues including the liver, epidermis, pancreatic islet cells, connective tissues, cardiac muscle and smooth muscle, and are also common in embryonic tissues. In the central nervous system, they are found in the ependyma and between neuroglial cells, and they form electrical synapses between some types of neurone, although this is rare in humans. Very recently, a second family of gap junctional proteins has been discovered, the pannexins. In humans, expression of pannexins has been most extensively studied in the nervous system (reviewed in Litvin et al 2006).
Other types of junction
Chemical synapses and neuromuscular junctions are specialized areas of intercellular adhesion where neurotransmitters secreted from a neuronal terminal gain access to specialized receptor molecules on a recipient cell surface. They are described on pages 44 and 62, respectively.
Cell signalling
The signal may act over a long distance, e.g. endocrine signalling through the release of hormones into the bloodstream, or neuronal synaptic signalling via electrical impulse transmission along axons and subsequent release of chemical transmitters of the signal at synapses (p. 44) or neuromuscular junctions (p. 62). A specialized variation of endocrine signalling (neurocrine or neuroendocrine signalling) occurs when neurones or paraneurones (e.g. chromaffin cells of the suprarenal medulla) secrete a hormone into interstitial fluid and the bloodstream.
These different types of intercellular signalling mechanism are illustrated in Figure 1.6. For further reading, see Alberts et al (2002) and Pollard & Earnshaw (2007).
Signalling molecules and their receptors
The majority of signalling molecules (ligands) are hydrophilic. They cannot cross the plasma membrane of a recipient cell to effect changes intracellularly unless they first bind to a plasma membrane receptor protein. Ligands are mainly proteins (usually glycoproteins), polypeptides or highly charged biogenic amines. They include: classic peptide hormones of the endocrine system; cytokines, which are mainly of haemopoietic cell origin and involved in inflammatory responses and tissue remodelling (e.g. the interferons, interleukins, tumour necrosis factor, leukaemia inhibitory factor); polypeptide growth factors (e.g. the epidermal growth factor superfamily, nerve growth factor, platelet-derived growth factor, the fibroblast growth factor family, transforming growth factor beta and the insulin-like growth factors). Polypeptide growth factors are multifunctional molecules with more widespread actions and cellular sources than their names suggest. They and their receptors are commonly mutated or aberrantly expressed in certain cancers. The cancer-causing gene variant is termed a transforming oncogene and the normal (wild-type) version of the gene is a cellular oncogene or proto-oncogene. The activated receptor acts as a transducer to generate intracellular signals, which are either small diffusible second messengers (e.g. calcium, cyclic adenosine monophosphate or the plasma membrane lipid-soluble diacylglycerol), or larger protein complexes that amplify and relay the signal to target control systems. For further reading on growth factors and other signalling molecules, see Epstein (2003).
Receptor proteins
Cell surface receptor proteins are generally grouped according to their linkage to one of three intracellular systems: ion channel-linked receptors; G-protein-coupled receptors; receptors that link to enzyme systems. Other receptors do not fit neatly into any of these categories. All the known G-protein-coupled receptors belong to a structural group of proteins that pass through the membrane seven times in a series of serpentine loops. These receptors are thus known as seven-pass transmembrane receptors or, because the transmembrane regions are formed from α-helical domains, as seven-helix receptors. The most well-known of this large group of phylogenetically ancient receptors are the odorant-binding proteins of the olfactory system, the light-sensitive receptor protein, rhodopsin, and many of the receptors for clinically useful drugs. A comprehensive list of receptor proteins, their activating ligands and examples of the resultant biological function, is given in Pollard & Earnshaw (2007).
Intracellular signalling
A wide variety of small molecules carry signals within cells, conveying the signal from its source (e.g. activated plasma membrane receptor) to its target (e.g. the nucleus). These second messengers convey signals as fluctuations in local concentration, according to rates of synthesis and degradation by specific enzymes (e.g. cyclases involved in cyclic nucleotide (cAMP, cGMP) synthesis), or, in the case of calcium, according to the activities of calcium channels and pumps. Other, lipidic, second messengers such as phosphatidylinositol, derive from membranes and may act within the membrane to generate downstream effects. For further consideration of the complexity of intracellular signalling pathways, see Pollard & Earnshaw (2007).
Exocytosis and endocytosis
The process of endocytosis involves the internalization of vesicles derived from the plasma membrane. The vesicles may contain: engulfed fluids and solutes from the extracellular interstitial fluid (pinocytosis); larger macromolecules, often bound to surface receptors (receptor-mediated endocytosis); particulate matter, including microorganisms or cellular debris (phagocytosis). Pinocytosis generally involves small fluid-filled vesicles and is a marked property of capillary endothelium, e.g. where vesicles containing nutrients and oxygen dissolved in blood plasma are transported from the vascular lumen to the endothelial basal plasma membrane (see Fig. 6.11). Interstitial fluid containing dissolved carbon dioxide is also taken up by pinocytosis for simultaneous transportation across the endothelial cell wall in the opposite direction, for release into the bloodstream by exocytosis. This shuttling of pinocytotic vesicles is also termed transcytosis. Larger volumes of fluid are engulfed by dendritic cells, e.g. in the process of sampling interstitial fluids by macropinocytosis in immune surveillance for antigens (p. 79). Interstitial fluid is inevitably taken up during receptor-mediated endocytosis when ligands are internalized.
Receptor-mediated endocytosis, also known as clathrin-dependent endocytosis, is initiated at specialized regions of the plasma membrane known as clathrin-coated pits. Clathrin is a protein that cross-links adjacent adaptor protein (adaptin) complexes to form a basket-like structure, bending the membrane inwards into a hemisphere. Much, but not all, fluid-phase pinocytosis also utilizes clathrin-coated pits. Ligands such as the iron-transporting protein, transferrin, and the cholesterol-transporting low-density lipoprotein bind to their receptors, which cluster in clathrin-coated pits through an interaction with adaptins. The pits then invaginate and pinch off from the plasma membrane, internalizing both receptor and ligand. The processing of endocytic vesicles and their contents is described on p. 12. For further details of the molecular mechanisms of endocytosis, see Alberts et al (2002) or Pollard & Earnshaw (2007).
Phagocytosis
Phagocytosis is a property of many cell types, but is most efficient in cells specialized for this activity. The professional phagocytes of the body belong to the monocyte lineage of haemopoietic cells, in particular the tissue macrophages (p. 78). Other effective phagocytes are neutrophil granulocytes and most dendritic cells (p. 79), which are also of haemopoietic origin. Phagocytosis plays an important part in the immune defence system of the body, in which the amoeboid process of ingestion of organisms for nutrition has evolved into a mechanism for the clearance of microorganisms invading the body. Macrophages also ingest particulate material including inorganic matter, such as inhaled dust particles, in addition to debris from dead cells and protein aggregates such as immune complexes in the blood, airways, interstitial spaces and connective tissue matrices.
CYTOPLASM
Endoplasmic reticulum
Endoplasmic reticulum is a system of interconnecting membrane-lined channels within the cytoplasm (Fig. 1.7). These channels take various forms, including cisternae (flattened sacs), tubules and vesicles. The membranes divide the cytoplasm into two major compartments. The intramembranous compartment includes the space where secretory products are stored or transported to the Golgi complex and cell exterior. The extramembranous cytosol is made up of the colloidal proteins such as enzymes, carbohydrates and small protein molecules, together with ribosomes and ribonucleic acids, and elements of the cytoskeleton.
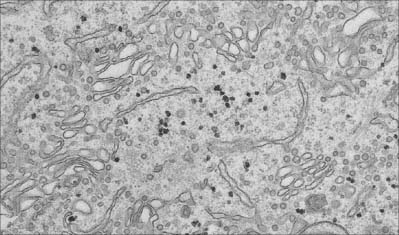
Fig. 1.7 Smooth endoplasmic reticulum with associated vesicles. The dense particles are glycogen granules.
(By courtesy of Rose Watson, Cancer Research UK.)
Structurally, the channel system can be divided into rough or granular endoplasmic reticulum, which has ribosomes attached to its outer cytosolic surface, and smooth or agranular endoplasmic reticulum, which lacks ribosomes. Functionally, the endoplasmic reticulum is compartmentalized into specialized regions with unique functions. For further reading see Levine & Rabouille (2005).
Rough endoplasmic reticulum
The rough endoplasmic reticulum, studded with ribosomes, is a site of protein synthesis (Fig. 1.8). Most proteins pass through its membranes and accumulate within its cisternae, although some integral membrane proteins, e.g. plasma membrane receptors, are inserted into the rough endoplasmic reticulum membrane, where they remain. After passage from the rough endoplasmic reticulum, proteins remain in membrane-bound cytoplasmic organelles such as lysosomes, become incorporated into new plasma membrane, or are secreted by the cell. Some carbohydrates are also synthesized by enzymes within the cavities of the rough endoplasmic reticulum and may be attached to newly formed protein (glycosylation). Vesicles are budded off from the rough endoplasmic reticulum for transport to the Golgi as part of the protein-targeting mechanism of the cell.
Smooth endoplasmic reticulum
The smooth endoplasmic reticulum (Fig. 1.7) is associated with carbohydrate metabolism and many other metabolic processes, including detoxification and synthesis of lipids, cholesterol and other steroids. The membranes of the smooth endoplasmic reticulum serve as surfaces for the attachment of many enzyme systems, e.g. the enzyme cytochrome P450, which is involved in important detoxification mechanisms and is thus accessible to its substrates, which are generally lipophilic. They also cooperate with the rough endoplasmic reticulum and the Golgi apparatus to synthesize new membranes; the protein, carbohydrate and lipid components are added in different structural compartments. Highly specialized types of endoplasmic reticulum are present in some cells. For example, in skeletal muscle cells, the smooth endoplasmic reticulum (sarcoplasmic reticulum) stores calcium ions, which are released into the cytosol to initiate contraction after stimulation initiated by a motor neurone at the neuromuscular junction (p. 62).
Ribosomes
A typical cell contains millions of ribosomes. They may be solitary, relatively inactive structures, or may form groups (polyribosomes or polysomes) attached to messenger RNA (mRNA), which they translate during protein synthesis. Polysomes may be attached to the membranes of the rough endoplasmic reticulum (see Fig. 1.8) or may lie free in the cytosol, where they synthesize proteins for use outside the system of membrane compartments, including enzymes of the cytosol and cytoskeletal proteins. Some of the cytosolic products include proteins that can be inserted directly into (or through) membranes of selected organelles, such as mitochondria and peroxisomes.
In a mature polysome, all the attachment sites of the mRNA are occupied as ribosomes move along it, synthesizing protein according to its nucleic acid sequence. Consequently, the number of ribosomes in a polysome indicates the length of the mRNA molecule and hence the size of the protein being made. The two subunits have separate roles in protein synthesis. The 40S subunit is the site of attachment and translation of mRNA. The 60S subunit is responsible for the release of the new protein and, where appropriate, attachment to the endoplasmic reticulum via an intermediate docking protein that directs the newly synthesized protein through the membrane into the cisternal space.
Golgi apparatus (Golgi complex)
Ultrastructurally, the Golgi apparatus is a membranous organelle (Fig. 1.8) consisting of a stack of several flattened membranous cisternae, together with clusters of vesicles surrounding its surfaces. Seen in vertical section, it is often cup-shaped. Small transport vesicles from the rough endoplasmic reticulum, generated by a process of budding and pinching off, are received at one face of the Golgi stack, the convex cis-face (entry or forming surface). Here, they deliver their contents to the first cisterna in the series by membrane fusion. From the edges of this cisterna, the protein is transported to the next cisterna by vesicular budding and then fusion, and this process is repeated until the final cisterna at the concave trans face (exit or condensing surface) is reached. Here, larger vesicles are formed for delivery to other parts of the cell.
Endosomes, lysosomes, proteasomes and peroxisomes
The endosome system of vesicles originates in small endocytic vesicles (clathrin-coated vesicles and caveolae) or larger phagosomes and macropinocytic vesicles taken up by the cell from the exterior. Clathrin-dependent endocytosis occurs at specialized patches of plasma membrane called coated pits; this mechanism is also used to internalize ligands bound to surface receptor molecules and is also termed receptor-mediated endocytosis. Caveolae (little caves) are structurally distinct vesicles most widely used by endothelial and smooth muscle cells, where they are involved in transcytosis, signal transduction and possibly other functions. For further reading, see Pollard & Earnshaw (2007).
Late endosomes
After a brief period in the early endosomes, materials can be passed on to late endosomes, which are a more deeply placed set of tubules, vesicles or cisternae. Late endosomes receive lysosomal enzymes via vesicles (small lysosomes) transported from the Golgi apparatus. The pH of late endosomes is low (about 5.0) and this activates lysosomal acid hydrolases to degrade the endosomal contents. The products of hydrolysis are either passed through the membrane into the cytosol, or may be retained in the endosome. Late endosomes (Fig. 1.8) may grow considerably in size by vesicle fusion to form multivesicular bodies and the enzyme concentration may increase greatly to form the large, dense classic lysosomes described by de Duve (1963). However, such large organelles do not appear in all cells, perhaps because late endosomes often deal very rapidly with endocytosed material.
Lysosomes
Lysosomes are dense, spheroidal, membrane-bound bodies 80–800 nm in diameter (Fig. 1.8, Fig. 1.9), often with complex inclusions of material undergoing hydrolysis (secondary lysosomes). They contain acid hydrolases able to degrade a wide variety of substances. To date, more than 40 lysosomal enzymes have been described, including proteases, lipases, carbohydrases, esterases and nucleases. The enzymes are heavily glycosylated, and are maintained at a low pH by proton pumps in the lysosomal membranes.
Material that has been hydrolysed within late endosomes and lysosomes may be completely degraded to soluble products, e.g. amino-acids, which are recycled through metabolic pathways. However degradation is usually incomplete, and some debris remains. A debris-laden vesicle is called a residual body or tertiary lysosome (Fig. 1.8B), and may be passed to the cell surface, where it is ejected by exocytosis; alternatively, it may persist inside the cell as an inert residual body. Considerable numbers of residual bodies can accumulate in long-lived cells, often fusing to form larger dense vacuoles with complex lamellar inclusions. As their contents are often darkly pigmented, this may change the colour of the tissue, e.g. in neurones the end-product of lysosomal digestion, lipofuscin (neuromelanin or senility pigment), gives ageing brains a brownish-yellow colouration.
Lysosomal enzymes may also be secreted – often as part of a process to alter the extracellular matrix, as in osteoclast erosion of bone (p. 88). Abnormal release of enzymes can cause tissue damage, as in certain types of arthritis. Some drugs, e.g. cortisone, can stabilize lysosomal membranes and may therefore inhibit many lysosomal activities, including the secretion of enzymes, and their fusion with phagocytic vesicles.
Mitochondria
Mitochondria are membrane-bound organelles (Fig. 1.9). They are the principal source of chemical energy in most cells. Mitochondria are the site of the citric acid (Krebs’, tricarboxylic acid) cycle and the electron transport (cytochrome) pathway by which complex organic molecules are finally oxidized to carbon dioxide and water. This process provides the energy to drive the production of ATP from ADP and inorganic phosphate (oxidative phosphorylation). The various enzymes of the citric acid cycle are located in the mitochondrial matrix, whereas those of the cytochrome system and oxidative phosphorylation are localized chiefly in the inner mitochondrial membrane. It is now known that in many tissues, especially smooth muscle, mitochondria also play an important role in cell signalling, especially intracellular calcium homeostasis. They are also major producers of reactive oxygen species and oxidant stress, and are involved in activation of apoptosis.
The mitochondrial matrix is an aqueous environment. It contains a variety of enzymes, and strands of mitochondrial DNA with the capacity for transcription and translation of a unique set of mitochondrial genes (mitochondrial mRNAs and transfer RNAs, mitochondrial ribosomes with rRNAs). The DNA forms a closed loop, about 5 μm across; several identical copies are present in each mitochondrion. The ratio between its bases differs from that of nuclear DNA, and the RNA sequences also differ in the precise genetic code used in protein synthesis. At least 13 respiratory chain enzymes of the matrix and inner membrane are encoded by the small number of genes along the mitochondrial DNA. The great majority of mitochondrial proteins are encoded by nuclear genes and made in the cytosol, then inserted through special channels in the mitochondrial membranes to reach their destinations. Their membrane lipids are synthesized in the endoplasmic reticulum.
Mitochondria are distributed within a cell according to regional energy requirements, e.g. near the bases of cilia in ciliated epithelia, in the basal domain of the cells of proximal convoluted tubules in the renal cortex (where considerable active transport occurs) and around the proximal end of the flagellum in spermatozoa. They may be involved with tissue-specific metabolic reactions, e.g. various urea-forming enzymes in liver cell mitochondria. Moreover, a number of genetic diseases of mitochondria affect particular tissues exclusively, e.g. mitochondrial myopathies (skeletal muscle) and mitochondrial neuropathies (nervous tissue). For further information see Graff et al (2002).
Cytosolic organelles
Cytoskeleton
The catalogue of cytoskeletal structural proteins is extensive and still increasing. The major filamentous structures found in non-muscle cells are microfilaments (actin), microtubules (tubulin), and intermediate filaments (assemblies of cell type-specific intermediate filament proteins). Other important components are proteins that bind to the principal filamentous types to link them together or to generate movement. These include actin-binding proteins such as myosin, which in some cells can assemble into thick filaments, and microtubule-associated proteins. Pathologies involving cytoskeletal abnormalities are reviewed in Ramaekers & Bosman (2004).
Actin filaments (microfilaments)
Actin filaments are flexible filaments with a width of 6–8 nm (Fig. 1.10), and a solid cross-section. Within most cell types, actin constitutes the most abundant protein and in some motile cells its concentration may exceed 200 μM (10 mg protein per ml cytoplasm). The filaments are formed by the ATP-dependent polymerization of actin monomer (with a molecular mass of 43 kDa) into a characteristic linear form in which the subunits are arranged in a single tight helix with a distance of 13 subunits between turns. The polymerized form is termed F-actin (fibrillar actin) and the unpolymerized form is G-actin (globular actin). Each monomer has an asymmetric structure. When monomers polymerize, they confer a defined polarity on the filament: the plus end favours monomer addition, and the minus end favours monomer dissociation. Myosins bind to filamentous actin at an angle to give the appearance of a series of arrowheads pointing towards the minus end of the filament, and the barbs point towards the plus end. There is a dynamic equilibrium between G-actin and F-actin: in most cells about 50% of the actin is estimated to be in the polymerized state.
Actin-binding proteins
Other classes of actin-binding protein link the actin cytoskeleton to the plasma membrane either directly or indirectly through a variety of membrane-associated proteins. The latter may also create links via transmembrane proteins to the extracellular matrix. Best known of these is the family of spectrin-like molecules, which can bind to actin and also to each other and various membrane-associated proteins to create supportive networks beneath the plasma membrane. Defects in such molecules are linked to a number of inherited diseases (reviewed in Bennett & Healy 2008). Spectrin is found in erythrocytes, and closely related molecules are present in many other cells; for instance, fodrin is found in nerve cells, and dystrophin occurs in muscle cells, linking the contractile apparatus with the extracellular matrix via integral membrane proteins. Proteins such as ankyrin (which also binds actin directly), vinculin, talin, zyxin and paxillin connect actin-binding proteins to integral plasma membrane proteins such as integrins (directly or indirectly), and thence to focal adhesions. Myosin I and other unconventional myosins connect actin filaments to membranous structures, including the plasma membrane and transport vesicle membranes. Tropomyosin, an important regulatory protein of muscle fibres, is also present in non-muscle cells, where its function may be primarily to stabilize actin filaments against depolymerization. For further reading see Pollard & Earnshaw (2007).
Intermediate filaments
Intermediate filaments are about 10 nm thick and formed by a heterogeneous group of filamentous proteins. They are found in different cell types and are often present in large numbers, either where structural strength is needed (Fig. 1.10B,C), or to provide scaffolding for the attachment of other structures. It is likely that more complex, non-mechanical functions of intermediate filaments, with implications for human disease, remain to be discovered (see Toivola et al 2005). Intermediate filaments of different molecular classes are characteristic of particular tissues or states of maturity. They are therefore important indicators of the origins of cells or levels of differentiation, and are of considerable value in histopathology.
Of the different classes of intermediate filaments, keratin (cytokeratin) proteins are found in epithelia, where keratin filaments are always composed of equal ratios of types I (acidic) and II (basic to neutral) keratins. About 20 types of each of the acidic and basic/neutral keratin proteins are known. Within the epidermis, expression of keratin heterodimer combinations changes as keratinocytes mature during their transition from basal to superficial layers. Genetic abnormalities of keratins are known to affect the mechanical stability of epithelia. For example, the disease epidermolysis bullosa simplex causes lysis of epidermal basal cells and blistering of the skin after mechanical trauma. It is caused by defects in genes encoding keratins 5 and 14, which produce cytoskeletal instability and thus cellular fragility in the basal cells. When keratins 1 and 10 are affected, cells in the prickle cell layer of the epidermis lyse, and this produces the intraepidermal blistering of epidermolytic hyperkeratosis. For a review, see Porter & Lane (2003).
Vimentins occur in mesenchyme-derived cells of connective tissue, desmins in muscle cells, glial fibrillary acidic protein in glial cells, and peripherin in peripheral axons. Neurofilaments are a major cytoskeletal element in neurones, particularly in axons (Fig. 1.10C), where they are the dominant protein. They are heteropolymers of low, medium and high molecular weight neurofilament proteins; the low molecular weight form is always present in combination with either the medium- or the high-molecular weight neurofilament. Abnormal accumulations of neurofilaments (neurofibrillary tangles) are characteristic features of a number of neuropathological conditions.
Microtubules
Microtubules are polymers of tubulin with the form of hollow, relatively rigid cylinders, approximately 25 nm in diameter and of varying length (up to 70 μm in spermatozoan flagella). They are present in most cell types, and are particularly abundant in neurones (Fig. 1.10C), leukocytes and blood platelets. They are the predominant constituent of the mitotic spindles of dividing cells. They also form part of the structure of cilia, flagella and centrioles.
Centrioles, centrosomes and basal bodies
Centrioles are microtubular cylinders 0.2 μm in diameter and 0.4 μm long (Fig. 1.11). They are formed by a ring of nine microtubule triplets linked by a number of other proteins. At least two centrioles occur in all animal cells that are capable of mitotic division (eggs, which undergo meiosis instead of mitosis, lack centrioles). They usually lie close together, at right angles or, most usually, at an oblique angle to each other (an arrangement often termed a diplosome), within the centrosome, a densely filamentous region of cytoplasm at the centre of the cell. The centrosome is the major microtubule-organizing centre of most cells; it is the site at which new microtubules are formed and the mitotic spindle is generated during cell division. Centriole biogenesis is a complex process that takes more than a single cell cycle to complete. At the beginning of the S phase (DNA replication phase) of the cell cycle, a new daughter centriole forms at right angles to each separated maternal centriole. Each mother–daughter pair forms one pole of the next mitotic spindle, and the daughter centriole becomes fully mature only as the progeny cells are about to enter the next mitosis. Because centrosomes are microtubule organizing centres, they lie at the centre of a network of microtubules all of which have their minus ends proximal to the centrosome. The association of membrane vesicles with dynein motors means that certain membranes (including the Golgi apparatus) concentrate near the centrosome. This is convenient, as the microtubules provide a means of targeting Golgi vesicular products to different parts of the cell.
Cell surface projections
Cilia and flagella
A cilium or flagellum consists of a shaft (0.25 μm diameter) constituting most of its length, a tapering tip and a basal body at its base, which lies within the surface cytoplasm of the cell (Fig. 1.12). Other than at its base, the entire structure is covered by plasma membrane. The core of the cilium is the axoneme, a cylinder of nine microtubule doublets that surrounds a central pair of single microtubules (Fig. 1.12). However, there is a class of cilia (primary cilia and nodal cilia) that are composed of nine microtubule doublets and no central microtubules.
When a cilium bends, the microtubules do not change in length, but slide on one another. The dynein arms of peripheral doublets slant towards the base of the cilium from their attached ends. Dynein has an ATPase activity, which causes mutual sliding of adjacent doublets by initially attaching sideways to the next pair, then swinging upwards towards the tip of the cilium. There is a group of genetic diseases (reviewed in Afzelius 2004) in which cilia beat either ineffectively or not at all, e.g. Kartagener’s immotile cilia syndrome. Affected cilia exhibit various ultrastructural defects, such as a lack of dynein arms or missing spokes. Patients with this syndrome suffer various respiratory problems caused by the accumulation of particles in the lungs; males are typically sterile because of the loss of sperm motility, and 50% have an alimentary tract that is a mirror image of the usual pattern (situs inversus) – i.e. it rotates in the opposite direction during early development.
Microvilli
Microvilli are finger-like cell surface extensions usually 0.1 μm in diameter and up to 2 μm long (Fig. 1.13). When arranged in a regular parallel series, they constitute a striated border, as typified by the absorptive surfaces of the epithelial enterocytes of the small intestine. When they are less regular, as in the gallbladder epithelium and proximal kidney tubules, the term brush border is used.
NUCLEUS
The nucleus (Figs 1.1, 1.2) is generally the largest intracellular structure and is usually spherical or ellipsoid in shape, with a diameter of 3–10 μm. Histological stains used to identify nuclei in tissue sections mainly detect the acidic molecules of deoxyribonucleic acid (DNA), which are largely confined to the nucleus.
Nuclear membrane
A special class of intermediate filaments known as lamins is associated with the inner surface of the nuclear membrane. The lamins form a dense meshwork beneath the membrane, the nuclear lamina. The lamin filaments cross each other at right angles to create an irregular anastomosing network that covers the interior surface of the nuclear membrane. In so doing, they reinforce the nuclear membrane mechanically, determine the shape of the nucleus and provide a binding site for a range of proteins that anchor chromatin. Nuclear lamin A, with over 350 mutations, is the most mutated protein linked to human disease. Lamin A mutations cause a surprisingly wide range of diseases, from progeria to various dystrophies (reviewed in Mattout et al 2006 and Pollard and Earnshaw 2007).
The transport of molecules between the nucleus and the cytoplasm occurs via specialized nuclear pore structures that perforate the nuclear membrane (Fig. 1.14A). They act as highly selective directional molecular filters, permitting proteins such as histones and gene regulatory proteins (which are synthesized in the cytoplasm but function in the nucleus) to enter the nucleus, and molecules that are synthesized in the nucleus but destined for the cytoplasm (e.g. ribosomal subunits, transfer RNAs and messenger RNAs), to leave the nucleus.
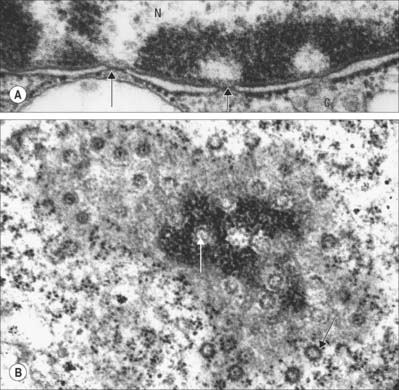
Fig. 1.14 A, Nuclear envelope with nuclear pores (arrowed) in transverse section, showing the continuity between the inner and outer phospholipid layers of the envelope on either side of the pore. The fine ‘membrane’ appearing to span the pore is formed by proteins of the pore complex. Note that the chromatin is less condensed in the region of nuclear pores. Nucleus (N); cytoplasm (C). B, Nuclear pores seen ‘en face’ as spherical structures (arrows) in a tangential section through the nuclear envelope. The appearance of the envelope varies in electron density as the plane of section passes through different regions of the curved double membrane, which is interrupted at intervals by pores through the envelope (see also Fig. 1.1). The surrounding cytoplasm with ribosomes is less electron-dense. Human tissues.
Ultrastructurally, nuclear pores appear as disc-like structures with an outer diameter of 130 nm and an inner pore with an effective diameter for free diffusion of 9 nm (Fig. 1.14B). The nuclear membrane of an active cell is bridged by up to 4000 such pores. The nuclear pore complex has an octagonal symmetry and is formed by an assembly of more than 50 proteins, the nucleoporins. The inner and outer nuclear membranes fuse around the pore complex (Fig. 1.14A). Nuclear pores are freely permeable to small molecules, ions and proteins up to about 17 kDa. Most proteins that enter the nucleus do so as complexes with specific transport receptor proteins known as importins. Importins shuttle back and forth between the nucleus and cytoplasm. Binding of the cargo to the importin requires a short sequence of amino acids known as a nuclear localization sequence (NLS), and can be either direct or via an adapter protein. Interactions of the importin with components of the nuclear pore move it together with its cargo through the pore by an energy-independent process still not understood. A complementary cycle functions in export of proteins and RNA molecules from the nucleus to the cytoplasm using transport receptors known as exportins. For further explanation, see Pollard & Earnshaw (2007).
Chromatin
DNA is organized within the nucleus in a DNA–protein complex known as chromatin. The protein constituents of chromatin are the histones and the non-histone proteins. Non-histone proteins are an extremely heterogeneous group that includes structural proteins, DNA and RNA polymerases and gene regulatory proteins. Histones are the most abundant group of proteins in chromatin, primarily responsible for the packaging of chromosomal DNA into its primary level of organization, the nucleosome. There are four core histone proteins: H2A, H2B, H3 and H4 that combine in equal ratios to form a compact octameric nucleosome core. A fifth histone, H1, is involved in further compaction of the chromatin. The DNA molecule (one per chromosome) winds twice around each nucleosome core, taking up 165 nucleotide pairs. This packaging organizes the DNA into a chromatin fibre 11 nm in diameter, and imparts to this form of chromatin the electron microscopic appearance of beads on a string, in which each bead is separated by a variable length of DNA, typically about 35 nucleotide pairs long. The nucleosome core region and one of the linker regions constitute the nucleosome proper, which is typically about 200 nucleotide pairs in length. However, chromatin rarely exists in this simple form and is usually packaged further into a 30 nm thick fibre, involving a single H1 histone per nucleosome, which interacts with both DNA and protein to impose a higher order of nucleosome packing. Usually, 30 nm fibres are further coiled or folded into larger domains. Individual domains are believed to decondense and extend during active transcription. In a typical interphase nucleus, euchromatin (nuclear regions that appear pale in appropriately stained tissue sections, or relatively electron-lucent in electron micrographs; Fig. 1.2) is likely to consist mainly of 30 nm fibres and loops, and contains the transcriptionally active genes. Transcriptionally active cells, such as most neurones, have nuclei that are predominantly euchromatic and often described as ‘open face’ nuclei.
Heterochromatin (nuclear regions that appear dark in appropriately stained tissue sections or electron-dense in electron micrographs) is characteristically located mainly around the periphery of the nucleus, except over the nuclear pores (Fig. 1.14A), and around the nucleolus (Fig. 1.2). It is a relatively compacted form of chromatin in which the histone proteins carry a specific set of post-translational modifications, including methylation at characteristic residues. This facilitates the binding of specific heterochromatin-associated proteins. Heterochromatin includes non-coding regions of DNA, such as centromeric regions, which are known as constitutive heterochromatin. DNA that is inactivated (becoming resistant to transcription) in some cells as they differentiate during development or cell maturation contributes to heterochromatin, and is known as facultative heterochromatin. The inactive X chromosome in females is an example of facultative heterochromatin and can be identified in the light microscope as the deeply staining Barr body (drumstick chromosome), often located near the nuclear periphery.
In transcriptionally inactive cells, chromatin is predominantly in the condensed, heterochromatic state, and may comprise as much as 90% of the total. Examples of such cells are mature neutrophil leukocytes (in which the condensed chromatin is present in a multilobed, densely staining nucleus), and the highly condensed nuclei of orthochromatic erythroblasts (late-stage erythrocyte precursors). In most mature cells, a mixture of the two occurs, indicating that only a proportion of the DNA is being transcribed. A particular instance of this is seen in the mature B lymphocyte (plasma cell), in which much of the chromatin is in the condensed condition and is arranged in regular masses around the perimeter of the nucleus, producing the so-called ‘clock-face’ nucleus (Figs 4.6, 4.12). Although this cell is actively transcribing, much of its protein synthesis is of a single immunoglobulin type, and consequently much of its genome is in an inactive state.
Chromosomes and karyotypes
Each chromosomal DNA molecule contains a number of specialized nucleotide sequences that are associated with its maintenance. One is the centromere. During mitosis, a disc-shaped structure composed of a complex array of proteins, the kinetochore, forms as a substructure of the centromeric region of DNA in order to attach it to the microtubular spindle. Another sequence, the telomere, defines the end of each chromosomal DNA molecule. Telomeres consist of hundreds of repeats of the nucleotide sequence (TTAGGG)n. The very ends of the chromosomes cannot be replicated by the same DNA polymerase as the rest of the chromosome, and are maintained by a specific enzyme called telomerase which contains an RNA subunit which acts as the template for lengthening the TTAGGG repeats. Thus telomerase is a specialized type of polymerase known as a reverse transcriptase that turns sequences in RNA back into DNA. The number of tandem repeats of the telomeric DNA sequence varies. It appears to shorten with successive cell divisions, because telomerase activity reduces or is absent in differentiated cells with a finite lifespan. It is believed that this mechanism contributes to regulation of cell senescence and may protect against proliferative disorders, including cancer (reviewed in Flores et al 2006).
Classification of human chromosomes
Lymphocytes separated from blood samples, or cells taken from other tissues, are used as a source of chromosomes. Diagnosis of fetal chromosome patterns is generally carried out on samples of amniotic fluid containing fetal cells aspirated from the uterus by amniocentesis, or on a small piece of chorionic villus tissue removed from the placenta. Whatever their origin, the cells are cultured in vitro and stimulated to divide by treatment with agents that stimulate cell division. Mitosis is interrupted at metaphase with spindle inhibitors. The chromosomes are dispersed by first causing the cells to swell in a hypotonic solution, then the cells are gently fixed and mechanically ruptured on a slide to spread the chromosomes. They are subsequently stained in various ways to allow the identification of individual chromosomes by size, shape and distribution of stain (Fig. 1.15). General techniques show the obvious landmarks, e.g. lengths of arms and positions of constrictions. Banding techniques demonstrate differential staining patterns, characteristic for each chromosome type. Fluorescence staining with quinacrine mustard and related compounds produces Q bands, and Giemsa staining (after treatment that partially denatures the chromatin) gives G bands (Fig. 1.15A). Other less widely used methods include: reverse-Giemsa staining, in which the light and dark areas are reversed (R bands); the staining of constitutive heterochromatin with silver salts (C-banding); T-banding to stain the ends (telomeres) of chromosomes. Collectively, these methods permit the classification of chromosomes into numbered autosomal pairs in order of decreasing size, from 1 to 22, plus the sex chromosomes.
A summary of the major classes of chromosomes is given below:
Group | Features |
---|---|
1–3 (A) | Large metacentric chromosomes |
4–5 (B) | Large submetacentric chromosomes |
6–12 + X (C) | Metacentrics of medium size |
13–15 (D) | Medium-sized acrocentrics with satellites |
16–18 (E) | Shorter metacentrics (16) or submetacentrics (17,18) |
19–20 (F) | Shortest metacentrics |
21–22 + Y (G) | Short acrocentrics; 21, 22 with satellites, Y without |
Methodological advances in banding techniques improved the recognition of abnormal chromosome patterns. The use of in situ hybridization with fluorescent DNA probes specific for each chromosome (Fig. 1.15B) permits the identification of even very small abnormalities.
Nucleolus
Nucleoli are a prominent feature of an interphase nucleus (Fig. 1.2). They are the site of most of the synthesis of rRNA and assembly of ribosome subunits. Ultrastructurally, the nucleolus appears as a pale fibrillar region (non-transcribed DNA), containing dense fibrillar cores (sites of rRNA gene transcription) and granular regions (sites of ribosome subunit assembly) within a diffuse nucleolar matrix. Five pairs of chromosomes carry rRNA genes organized in clusters of tandemly repeated units on each chromosome. Each rRNA unit is transcribed individually and encodes a large precursor RNA that is processed to yield the 28S, 18S and 5.8S rRNA molecules. This processing takes place in the nucleolus, as does the processing of a number of other stable RNAs, including the RNA component of the signal recognition particle (SRP), which is essential for protein secretion. During mitosis the nucleolus breaks down. It reforms after nuclear envelope reformation in telophase, in a process associated with the onset of transcription in nucleolar organizing centres on each chromosome. The 28S, 18S and 5.8S rRNA molecules are assembled into their ribosomal subunits in the granular region of the nucleolus together with the 5S rRNA, which is not synthesized in the nucleolus. The newly formed ribosomal subunits are then translocated to the cytoplasm through the nuclear pores.
CELL DIVISION AND THE CELL CYCLE
Patterns and rates of cell division within tissues vary considerably. In many epithelia, such as the crypts between intestinal villi, the replacement of damaged or effete cells by division of stem cells can be rapid. Rates of cell division may also vary according to demand, as occurs in the healing of wounded skin, in which cell proliferation increases to a peak and then returns to the normal replacement level (see Ch. 7). The rate of cell division is tightly coupled to the demand for growth and replacement. Where this coupling is faulty, tissues either fail to grow or replace their cells, or they can overgrow, producing neoplasms.
Cell cycle progression is driven in part by changes in the activity of cyclin-dependent protein kinases, CDKs (protein kinases which are activated by binding of a cyclin subunit). Each cell cycle stage is characterized by the activity of one or more CDK-cyclin pairs. Transitions between cell cycle stages are triggered by highly specific proteolysis of the cyclins and other key components. The targets for proteolysis are marked for destruction by E3 ubiquitin ligases, which decorate them with polymers of the small protein ubiquitin, a sign for recognition by the proteasome. To give one example, the transition from G2 to mitosis is driven by activation of CDK1 by its partners the A- and B-type cyclins: the characteristic changes in cellular structure that occur as cells enter mitosis are largely driven by phosphorylation of proteins by active CDK1-cyclin A and CDK1-cyclin B (further details are beyond the scope of this book). Cells exit from mitosis when the anaphase promoting complex/cyclosome (APC/C), an E3 ubiquitin ligase, marks the cyclins for destruction. The APC/C also triggers for destruction another protein whose function is to protect the cohesion between sister chromatids. An analogous process of protein phosphorylation coupled with targeted destruction drives the transition between G1 and S phase as cells commit to another cycle of proliferation.
There are important checkpoints in the cell cycle at which progress will be arrested if, for instance, DNA replication or mitotic spindle assembly and chromosome attachment are incomplete. Negative regulation systems also operate to delay cell cycle progression when DNA has been damaged by radiation or chemical mutagens. Cells with checkpoint defects, such as loss of the protein p53, which is a major negative control element in the division cycle of all cells, are commonly associated with the development of malignancy. Cells lacking one of the critical checkpoint functions are able to progress through the cycle carrying defects, thus increasing the probability that further abnormalities will accumulate in their progeny. The p53 gene is an example of a tumour suppressor gene. For further reading, see Blow & Tanaka (2005); Pollard & Earnshaw (2007).
MITOSIS AND MEIOSIS
Mitosis
New DNA is synthesized during the S phase of the cell cycle interphase. This means that the amount of DNA in diploid cells has doubled to the tetraploid value by the onset of mitosis, although the chromosome number is still diploid. During mitosis, this amount is halved between the two daughter cells, so that DNA quantity and chromosome number are diploid in both cells. The cellular changes that achieve this distribution are conventionally divided into four phases called prophase, metaphase, anaphase and telophase (Fig. 1.16, Fig. 1.17).
Anaphase
By the end of metaphase every chromosome consists of a pair of sister chromatids attached to opposing spindle poles by bundles of microtubules associated with the centromeres. The onset of anaphase begins with the proteolytic cleavage of a key subunit of a complex known as cohesin, which holds the replicated sister chromatids together. This cleavage releases the cohesion between sister chromatids, which each then move towards opposite spindle poles as the microtubule bundles attached to the centromeres shorten and move polewards. At the end of anaphase the sister chromatids are grouped at either end of the cell, and both clusters are diploid in number. An infolding of the cell equator begins, and deepens during telophase as the cleavage furrow.
Meiosis
There are two cell divisions during meiosis (Fig. 1.18). Details of this process differ at a cellular level for male and female lineages.
Meiosis I
Prophase I
Meiotic prophase I is a long and complex phase that differs considerably from mitotic prophase and is customarily divided into five substages, called leptotene, zygotene, pachytene, diplotene and diakinesis (see Pollard & Earnshaw 2007).
CELL DIFFERENTIATION
A cell may be committed to a particular differentiated fate without manifesting its commitment until later. Once switched in this way, cells are not usually able to revert to an earlier stage of commitment to a differentiation pathway, so that an irreversible repression of some gene sequences must have occurred. Differentiation signals include interactions between cells that are mediated by diffusible signalling molecules elaborated by one cell and detected by another, and by contactmediated signalling (such as Delta–Notch signalling). The latter is particularly important in establishing boundaries between different cell populations in development.
There are few instances of the transdifferentiation of one differentiated cell type into another (metaplasia, see Ch. 2), but there is evidence that stem cells in the developing embryo and in certain mature tissues (e.g. bone marrow) may have the potential to differentiate into more diverse phenotypes than was once believed. This plasticity depends on environmental cues and offers the prospect of engineering tissues for clinical therapy. For further reading, see Alberts et al (2002).
APOPTOSIS
The morphological changes exhibited by necrotic cells are very different from those seen in apoptotic cells (Fig. 1.19). Necrotic cells swell and subsequently rupture; the resulting debris may induce an inflammatory response (see Ch. 4, Ch. 7). Apoptotic cells shrink, their nuclei and chromosomes fragment, forming apoptotic bodies, and their plasma membranes undergo conformational changes that act as a signal to local phagocytes. The dead cells are removed rapidly, and as their intracellular contents are not released into the extracellular environment, inflammatory reactions are avoided; the apoptotic fragments also stimulate macrophages to release anti-inflammatory cytokines.
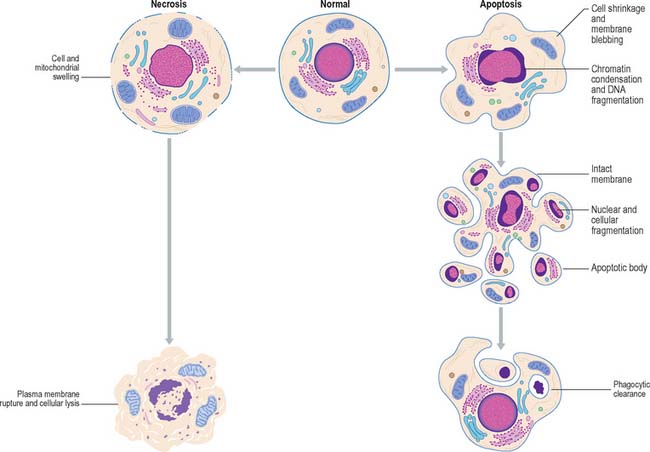
Fig. 1.19 Comparison between the changes in cellular structure during cell death by apoptosis and necrosis.
Subversion of the apoptotic response is a key characteristic of many cancer cells. Thus the tumour suppressor gene p53 (which functions in cell-cycle control, regulation of apoptosis and the maintenance of genetic stability), is mutated in about 50% of all human cancers. For further details, see Pollard & Earnshaw (2007).
Afzelius BA. Cilia-related diseases. J Pathol. 2004;204:470-477.
Alberts B, Johnson A, Lewis J, Raff M, Roberts K, Walter P. Molecular Biology of the Cell, 4th edn. New York: Garland Press, 2002.
Bennett V, Healy J. Organising the fluid membrane bilayer: diseases linked to spectrin and ankyrin. Trends Mol Med. 2008;14:28-36.
Blow JJ, Tanaka TU. The chromosome cycle: coordinating replication and segregation. EMBO Reports. 2005;6:1028-1034.
de Duve C. The lysosome. Sci Am. 1963;208:64-72.
Epstein RJ. Human Molecular Biology. In An Introduction to the Molecular Basis of Health and Disease. Cambridge: Cambridge University Press; 2003.
Reviews signalling molecules and their receptors in the context of human disease..
Flores I, Benetti R, Blasco MA. Telomerase regulation and stem cell behaviour. Curr Op Cell Biol. 2006;18:1-7.
Graff C, Bui T-H, Larsson N-G. Mitochondrial diseases. Best Pract Res Clin Obstet Gynaecol. 2002;16:715-728.
Reviews clinical conditions related to the inheritance of maternal mitochondrial gene mutations..
Gumbiner BM. Regulation of cadherin-mediated adhesion in morphogenesis. Nature Rev Mol Cell Biol. 2005;6:622-634.
Levine T, Rabouilee C. Endoplasmic reticulum: one continuous network compartmentalized by extrinsic cues. Curr Op Cell Biol. 2005;17:362-368.
Litvin O, Tiunova A, Connell-Alberts Y, Panchin Y, Baranova A. What is hidden in the pannexin treasure trove: the sneak peek and the guesswork. J Cell Mol Med. 2006;10:613-634.
Mattout A, Dechat T, Adam SA, Goldman RD, Gruenbaum Y. Nuclear lamins, diseases and aging. Curr Op Cell Biol. 2006;18:335-341.
Morris R, Cox H, Mombelli E, Quinn P. Rafts, little caves and large potholes: how lipid structure interacts with membrane proteins to create functionally diverse membrane environments. Quinn PJ, editor. Subcellular Biochemistry. vol 37. New York: Kluwer Academic/Plenum Publishers; 2004:35-118.
Pollard TD, Earnshaw WC. Cell Biology. Philadelphia: Saunders, 2007.
Porter RM, Lane EB. Phenotypes, genotypes and their contribution to understanding keratin function. Trends Genet. 2003;19:278-285.
Ramaekers FCS, Bosman FT. The cytoskeleton and disease. J Pathol. 2004;204:351-505.
Toivola DM, Tao G-Z, Habtezion A, Liao J, Omary MB. Cellular integrity plus: organelle-related and protein-targeting functions of intermediate filaments. Trends Cell Biol. 2005;15:608-617.