CHAPTER 3 Architectural Design and Function of Human Back Muscles
Spinal muscles generate movements of the spine and provide the stability needed to protect vital anatomic structures. Muscles must work in coordination with the rest of the neuromusculoskeletal system (i.e., vertebrae, tendons, ligaments, and the nervous system) to provide these functions. Large movements of the head require appropriate muscle strength, vertebral geometry (e.g., facet joint orientation), ligament compliance, and neural control. The spinal muscles have been described as one of three subsystems (the others are the passive spinal column and neural control) that must work together to stabilize the spine.1
Muscle Architecture
Skeletal muscle is highly organized at the microscopic level, as evidenced by classic studies that have elucidated the properties of skeletal muscle fibers. With few exceptions, the arrangement of muscle fibers within and between muscles has received much less attention. The microscopic arrangement of muscle fibers relative to the axis of force generation is known as the architecture of a muscle.2 Although muscle fiber size (which is directly proportional to force generation) is relatively consistent among muscles of different sizes, architectural differences between muscles show much more variability and more strongly affect function. Muscle architecture is a primary determinant of muscle function, and understanding this structure-function relationship has great practical importance. This understanding not only clarifies the physiologic basis for production of force and movement but also provides a scientific rationale for surgery. Muscle architectural studies also provide guidelines for electrode placement during electromyographic measures of muscle activity, explain the mechanical basis of muscle injury during movement, and aid in the interpretation of histologic specimens obtained from muscle biopsies.
Basic Architectural Definitions
The various types of architectural arrangement are as numerous as the muscles themselves. For discussion purposes, we describe three general classes of muscle fiber architecture. Muscles composed of fibers that extend parallel to the force-generating axis of the muscle are described as having a parallel or longitudinal architecture (Fig. 3–1A). Muscles with fibers that are oriented at a single angle relative to the force-generating axis are described as having unipennate architecture (Fig. 3–1B). The angle between the fiber and the force-generating axis has been measured at resting length in mammalian muscles over different designs and varies from about 0 to 30 degrees. Most muscles fall into the third and most general category, multipennate muscles—muscles constructed of fibers that are oriented at several angles relative to the axis of force generation (Fig. 3–1C).
Experimental Determination of Skeletal Muscle Architecture
Quantitative studies of muscle architecture were pioneered by Gans and colleagues,2,3 who developed precise methodology for defining muscle architecture based on microdissection of whole muscles. The parameters usually included in an architecture analysis are muscle length, fiber or fascicle length, pennation angle (i.e., the fiber angle relative to the force-generating axis), and physiologic cross-sectional area (PCSA). Typically, muscles are chemically fixed in formalin to maintain fiber integrity during dissection. The muscles should be chemically fixed while attached to the skeleton to preserve their physiologic length, or physiologic length in the skeleton should be noted. After fixation, muscles are dissected from the skeleton, their mass is determined, and their pennation angle and muscle length are measured.
Pennation angle (θ) is measured by determining the average angle of the fibers relative to the axis of force generation of the muscle. Usually only the pennation angle of fibers on the superficial muscle surface is measured; however, this is only an estimate because pennation angles may vary from superficial to deep and from proximal to distal. This superficial to deep variation in pennation has been documented in several spinal muscles (see later). Although more sophisticated methods could be developed for measurement of pennation angle, it is doubtful they would provide a great deal more insight into muscle function because variations in pennation angle may not strongly affect function.2
Muscle length is defined as “the distance from the origin of the most proximal muscle fibers to the insertion of the most distal fibers.”4 Fiber length represents the number of sarcomeres in series, and experimental evidence suggests that muscle fiber length is proportional to fiber contraction velocity.3,5 Muscle length and fiber length are not the same because there is a variable degree of “stagger” seen in muscle fibers as they arise from and insert onto tendon plates (see Fig. 3–1B). Muscle fiber length can be determined only by microdissection of individual fibers from fixed tissues or by laborious identification of fibers by glycogen depletion on serial sections along the length of the muscle.6 Unless investigators are explicit when they refer to muscle fiber length, they are probably referring to muscle fiber bundle length (also known as fascicle length) because it is extremely difficult to isolate intact fibers, which run from origin to insertion, especially in mammalian tissue.6,7
Experimental studies of mammalian muscle suggest that individual muscle fibers do not extend the entire muscle length and may not even extend the entire length of a fascicle.6,7 Detailed studies of individual muscle fiber length have not been conducted in human spinal muscles, but studies in feline neck muscles illustrate that muscle fibers are often arranged in series, ending in tendinous inscriptions within the muscle or terminating intrafascicularly.8,9 Although the terms fiber length and fascicle length are often used interchangeably, technically they are identical only if muscle fibers span the entire length of a fascicle. In muscle architecture studies, bundles consisting of 5 to 50 fibers are typically used to estimate fiber length, which may be reported as either fiber length or fascicle length.
To permit such conclusions, fiber length measurements should be normalized to a constant sarcomere length, which eliminates fiber length variability owing to variation in fixation length. Fiber (or fascicle) lengths are usually normalized to the optimal sarcomere length, the length at which a sarcomere generates maximum force. This normalized length is referred to as optimal fiber (or fascicle) length and provides a reference value that can be related back to the physiologic length if the relationship between muscle length and joint position is noted. Based on measured architectural parameters and joint properties, the relationship between sarcomere length and joint angle can be calculated. Because sarcomere length strongly influences muscle force generation, an understanding of the relationship between sarcomere length change and movement has been used in many studies to provide added understanding of muscle design.10–14
Mechanical Properties of Muscles with Different Architectures
Comparison of Two Muscles with Different Physiologic Cross-Sectional Areas
Suppose that two muscles have identical fiber lengths and pennation angles but one muscle has twice the mass (equivalent to saying that one muscle has twice the number of fibers and twice the PCSA). Also suppose that the two muscles have identical fiber type distributions and that they generate the same force per unit area. The functional difference between these two muscles is shown in Figure 3–2. The muscle with twice the PCSA has an isometric length-tension curve with the same shape, but it is amplified upward by a factor of 2. The maximal tetanic tension (Po) of the larger muscle would be twice that of the smaller muscle. Similarly, comparison of isotonic force-velocity curves indicates that the differences between muscles would simply be an upward shift in Po for the larger muscle.
Comparison of Two Muscles with Different Fiber Lengths
If two muscles have identical PCSAs and pennation angles but fiber lengths that differ, the schematic in Figure 3–3 shows that the effect of increased fiber length is to increase muscle excursion and velocity. Peak force of the length-tension curves is identical between muscles, but the range of lengths over which the muscle generates active force is different. For the same reason that an increased fiber length increases active muscle range of the length-tension relationship, it results in an increase in the maximum velocity (Vmax) of the muscle. Experimental support for this concept was obtained indirectly through observations of the cat semitendinosus muscle:4 When the proximal semitendinosus head was activated, its Vmax was 224 mm/sec, whereas when only the distal semitendinosus head was activated, its Vmax was 424 mm/sec. When both heads were activated simultaneously, the whole muscle Vmax was 624 mm/sec, or the sum of the two velocities. The values for Vmax were proportional to the different lengths of the proximal and distal heads. These data indicate that the longer the fibers in series (equivalent to saying the greater number of sarcomeres in series), the greater the muscle contraction velocity. As expected, maximum isometric tension was essentially the same regardless of which activation pattern was used.
Interplay of Muscle Architecture and Moment Arms
In addition to its architecture, the potential moment generated by a muscle is influenced by its moment arm. Moment arm, the “mechanical advantage” of a muscle, is the distance from the line of action of a muscle to the joint axis of rotation and is directly related to a muscle’s change in length with joint rotation.15 In other words, the amount of muscle fiber length change that occurs as a joint rotates and, consequently, the range of joint angles over which the muscle develops active force depend on the muscle moment arm. This idea can be explained by comparing the situation in which two muscles with identical fiber lengths have different moment arms at a joint (Fig. 3–4). In the case in which the moment arm is greater, the muscle fibers change length much more for a given change in joint angle compared with a muscle with a shorter moment arm. As a result, the range of joint motion over which the muscle develops active force is smaller for the muscle with the larger moment arm despite the fact that the muscular properties of both muscles are identical.
The architectural design of a muscle and its placement in relation to the skeletal geometry are important determinants of its function. Although muscles with longer fibers can generate force over a greater range of lengths than muscles with shorter fibers (see Fig. 3–3A), this does not indicate that muscles with longer fibers are associated with joints that have larger ranges of motion. Muscles that appear to be designed for speed based on their long fibers may not actually produce large joint velocities if they are placed in the skeleton with a very large moment arm because joint excursion and joint angular velocity are inversely related to moment arm. A large moment arm results in a large joint moment, so that the muscle would be highly suited for torque production but at low angular velocities. Similarly, a muscle that appears to be designed for force production because of the large PCSA, if placed in position with a very small moment arm, may actually produce high joint excursions or angular velocities. Differences between muscle-joint systems require complete analysis of joint and muscular properties. These interrelated concepts of architecture and moment arm (gross anatomy) must be considered when examining the design and function of spinal muscles.
Anatomy and Architecture of Spinal Musculature
Intrinsic Spinal Muscles Found in the Lumbar, Thoracic, or Cervical Spine
Intrinsic muscles of the spine are dominated by the erector spinae, a group of interdigitated muscles that spans the entire length of the spine, from the sacrum and iliac crest to the skull. Another important group of muscles, the multifidus, are shorter and deeper and are described in more detail later. In the thoracolumbar region, the erector spinae and multifidus muscles constitute the bulk of the spinal musculature. These two distinct functional units have large differences in innervation that probably result in significant functional differences,16 although the detailed biomechanical function of these groups remains only partially elucidated.17 Lying deep to the multifidus are even smaller muscles, the rotators, interspinales, and intertransversarii. The cervical region is composed of other intrinsic muscles unique to it (see later).
The erector spinae are commonly considered to be composed of three muscles; from medial to lateral, they are the spinalis, longissimus, and iliocostalis. The anatomy and architecture of these muscles vary among different levels of the spine. The words “lumborum,” “thoracis,” “cervicis,” and “capitis” are appended to the muscle name to describe the anatomy more accurately. Although there are varying definitions of the composition of the erector spinae, a study by MacIntosh and Bogduk17 provides the most comprehensive descriptive anatomy of the lumbar erector spinae, and Delp and colleagues18 provided the first architectural measurements of these muscles. The continuation of the erector spinae in the cervical region was discussed briefly by Kamibayashi and Richmond.19
The spinalis muscle is the most medial division of the erector spinae. MacIntosh and Bogduk17 described the spinalis as mostly aponeurotic in the lumbar region, but Delp and colleagues18 obtained architecture measurements from the spinalis in the thoracic region (Table 3–1). The spinalis is generally absent in the cervical region.
Caudal to rostral, the longissimus consists of the longissimus thoracis, cervicis, and capitis; the longissimus thoracis is divided into lumbar and thoracic portions. The lumbar fascicles of the longissimus thoracis (longissimus thoracis pars lumborum) are composed of five bands that arise from the lumbar transverse processes and attach in a caudal fashion onto the iliac crest (Fig. 3–5A). Each band arising from vertebra L1 to L4 is actually a small fusiform muscle that has an elongated and flattened caudal tendon of insertion. Bands from more rostral levels attach more medially on the iliac crest. The juxtaposition of these caudally located tendons form the lumbar intermuscular aponeurosis (see LIA in Fig. 3–5B).
Fascicles of the thoracic component of longissimus thoracis (longissimus thoracis pars thoracis) arise from all thoracic transverse processes and most ribs and attach to lumbar spinous processes, the sacrum, or the ilium. These are long slender muscles with pronounced caudal tendons that juxtapose to form the strong erector spinae aponeurosis, which bounds the lumbar paraspinal muscles dorsally. In the upper thoracic and cervical region, the longissimus cervicis connects transverse processes of thoracic and cervical vertebrae, whereas the longissimus capitis originates on transverse processes and inserts on the mastoid process of the skull (Fig. 3–6).
The lumbar fascicles of the iliocostalis lumborum (iliocostalis lumborum pars lumborum) lie lateral to the longissimus thoracis muscles arising from the tip of the transverse processes of vertebrae L1 to L4 in the lumbar region and are composed of four small, broad bands (see Fig. 3–5C) that attach to the thoracolumbar fascia and the iliac crest. The thoracic fascicles of the iliocostalis lumborum (iliocostalis lumborum pars thoracis) arise from ribs and attach to the iliac spine and crest, forming the lateral part of the erector spinae aponeurosis. In contrast to the more medially located longissimus thoracis, the caudal tendons are less prominent, giving the iliocostalis lumborum a much more fleshy appearance. Caudal to rib 10, the iliocostalis lumborum and longissimus thoracis lie side by side, forming the erector spinae aponeurosis. Rostral to rib 9 or 10, the iliocostalis thoracis separates the iliocostalis lumborum and longissimus thoracis. In the upper thoracic and cervical region, the iliocostalis cervicis connects the ribs to the transverse processes of cervical vertebrae.
MacIntosh and Bogduk17 measured muscle and tendon lengths in the thoracic portions of the longissimus thoracis and iliocostalis lumborum (Table 3–2). Detailed architecture of the lumbar erector spinae, including muscle tendon and fascicle length, sarcomere lengths, and PCSAs, has been measured (see Table 3–1).18 Fascicle lengths were found to be approximately 30% of muscle lengths in these muscles, and sarcomere lengths measured from cadavers in the supine position were generally shorter than the optimal length, which may imply that the erector spinae are capable of developing greater force in elongated positions (i.e., in flexion).
The lumbar multifidus muscles consist of multiple separate bands arising from each vertebral spinous process and lamina and inserting from two to four segments below the level of origin (Fig. 3–7B). The shortest fascicle of each muscle inserts onto the mammillary process of the vertebra located two segments caudal, whereas longer, more superficial fascicles insert sequentially onto subsequent vertebrae three or more segments lower (see Fig. 3–7). The shortest band of the multifidus arising from L1 inserts on L3, and subsequent bands insert sequentially on L4, L5, and the sacrum. Multifidus muscles arising from lower lumbar vertebrae consist of fewer fascicles because the number of vertebrae caudal to the origin decreases.
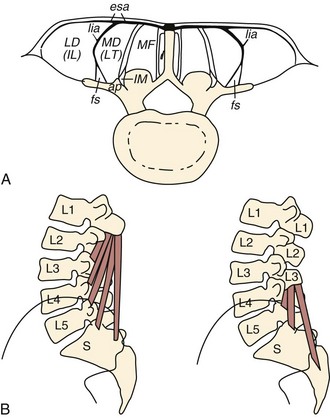
FIGURE 3–7 Schematic arrangement of multifidus muscle in cross section (A) and longitudinal section (B).
(From Bogduk N: A reappraisal of the anatomy of the human lumbar erector spinae. J Anat 131:525-540, 1980.)
All multifidus muscles that arise from a given level are innervated by the medial branch of the primary dorsal rami of the spinal nerve from a single segment (i.e., each band of multifidus muscle is innervated from a single dorsal ramus). In the cervical region, multifidus fascicles from the spinous processes and laminae of C2, C3, and C4 attach onto facet capsules of two adjacent vertebral articular processes from C4 to C7; fascicles from the spinous processes and laminae of C4 to C7 attach onto transverse processes of upper thoracic vertebrae.20 The principal action of the multifidus is extension, but the multisegmental nature of the muscle and the complex three-dimensional orientation in the craniocaudal and mediolateral directions renders this statement a gross oversimplification.21 The multifidus is not considered a prime mover of the spine; rather, its function is likely to produce small vertebral adjustments.
A study of the multifidus muscle revealed three major design factors that suit it well for stabilizing the lumbar spine.22 First, the architecture of the multifidus is highly pennated with fibers extending only about 20% of the length of the fascicles. Numerous muscle fibers are packed into a small volume, and even though the multifidus has a smaller mass compared with several other lumbar extensors, it is predicted to create the greatest lumbar extension force by a factor of 2 (see Table 3–1). Second, direct mechanical testing of the multifidus muscle cells and extracellular connective tissue revealed that although the multifidus fibers have the same mechanical properties as other limb muscles, the fiber bundles, which include extracellular connective tissue, are about twice as stiff as limb muscles. The multifidus has a high passive elastic capacity that would suit it for passively resisting flexion of the lumbar spine. Third, the multifidus muscle sarcomere length, measured intraoperatively, is relatively short when the spine is extended, suggesting that the muscle gets stronger as it gets longer. In other words, as the spine flexes, multifidus force increases, suiting it to restore spine angles toward neutral or more extended positions.
Deep to the multifidus are smaller muscles that span one or two vertebral segments. The rotatores attach from caudal transverse processes to the base of rostral spines one or two segments away. Rotators are prominent in the thoracic region, although some authors claim they exist in the lumbar region.23,24 MacIntosh and colleagues25 did not find any muscles deep to the lumbar multifidus. The interspinalis and intertransversarii, found in the lumbar and cervical regions, connect the spines and transverse processes of adjacent vertebrae.
Intrinsic Spinal Muscles Specific to the Cervical Spine
Because of different functional demands in the cervical spine (e.g., large head movements), this region has additional intrinsic muscles. Kamibayashi and Richmond19 provided details on neck muscle anatomy and quantitative architecture data of the neck muscles (Table 3–3).
Splenius Capitis and Cervicis
The splenius capitis originates at the spinous processes of the lower cervical and upper thoracic vertebrae and inserts on the skull near the mastoid process (see Fig. 3–6A). Contiguous, slightly deeper, and sometimes inseparable is the splenius cervicis, which originates on thoracic spinous processes and inserts on cervical transverse processes. Although the splenius capitis and splenius cervicis function in extension, lateral bending, and axial rotation, the splenius capitis is oriented more obliquely than the splenius cervicis, providing more axial rotation capacity for movements of the skull relative to the vertebrae. The fascicle lengths of the splenius capitis and splenius cervicis are similar, but their muscle tendon lengths are not similar; this occurs because the splenius capitis has short aponeuroses, whereas the splenius cervicis has long aponeuroses (Fig. 3–8A).19
Semispinalis Capitis and Cervicis
The semispinalis capitis originates on the articular processes of the lower cervical vertebrae and transverse processes of the upper thoracic vertebrae and inserts medially on the skull between the inferior and superior nuchal line (see Fig. 3–6A). The semispinalis capitis is characterized by complex patterns of internal tendon and tendinous inscriptions in the medial portion, whereas fascicles in the lateral portion are uninterrupted (see Fig. 3–8B).19 The semispinalis cervicis (deep to the semispinalis capitis) originates on thoracic transverse processes and inserts on cervical spinous processes from C2 to C5, with the bulk of its mass inserting on C2.
Longus Capitis and Colli
On the anterior side of the vertebral column, the longus capitis runs from the anterior surface of transverse processes to the baso-occiput (Fig. 3–9). Because it lies close to the vertebral bodies, it has only a small flexion moment arm; the superomedial orientation could provide ipsilateral rotation. Its counterpart, the longus colli, has a more complicated structure. Some fibers run vertically along the anterior vertebral bodies, other fibers run superolaterally from thoracic vertebral bodies to lower cervical transverse processes, and others run superomedially from transverse processes to the anterior vertebral bodies (see Fig. 3–9). Although all parts of the longus colli have small flexion moment arms, the superomedial and superolateral portions would have ipsilateral and contralateral rotation moment arms. The longus capitis and longus colli are also characterized by an aponeurosis covering much of the superficial surface, from which fascicles have long tendons that attach to the vertebrae (Fig. 3–10).19
Suboccipital Muscles
The suboccipital muscles span the region between C2 and the skull (see Fig. 3–6B). The rectus capitis posterior major and minor connect the spinous processes of C2 and C1 with the skull. The obliquus capitis superior is oriented in a superoinferior direction between the transverse process of C1 and the skull, and the obliquus capitis inferior runs primarily mediolaterally from the spinous process of C2 to the transverse process of C1. All four of these muscles can contribute to extension of the head with respect to the neck; in addition, the rectus capitis posterior major and the obliquus capitis inferior are oriented to produce ipsilateral rotation, and the lateral location of the obliquus capitis superior implies a lateral bending function. The obliquus capitis superior has an internal tendon on the deep surface that causes some fascicles to have large pennation angles.19 On the ventral side, the rectus capitis anterior and rectus capitis lateralis are very small muscles that connect the skull to C1, presumably with (small) moment arms for flexion and lateral bending.
Extrinsic Muscles Linking Vertebrae to the Pelvis
The quadratus lumborum attaches from the iliolumbar ligament and iliac crest onto the 12th rib and transverse processes of L1 to L4. It assists in lateral bending of the lumbar spine. The proximal component of the quadratus lumborum (i.e., the set of fascicles running from the iliac crest to the 12th rib and L1) has a larger moment arm for lateral flexion and has longer fascicles than the distal component of the muscle. Electromyographic evidence shows that the quadratus lumborum has a dominant role in spine stabilization.26
The psoas major attaches from the anterior surface of the transverse processes, the sides of vertebral bodies, and intervertebral discs of all lumbar vertebrae. Together with the iliacus, which arises from the ilium, they form the iliopsoas, which inserts on the lesser trochanter of the femur and is a major flexor of the thigh and trunk. Fascicles of the psoas generally have the same length, regardless of their level of origin. Because of their attachments to a common tendon, bundles from higher levels are more tendinous, whereas the bundle from L5 remains fleshy until it joins the common tendon.27
The psoas is the largest muscle in cross section at the lower levels of the lumbar spine.28 Biomechanical analysis shows that the psoas has the potential to flex the lumbar spine laterally, generate compressive forces that increase stability, and create large anterior shear forces at L5 to S1.29 If the psoas were designed for lumbar spine motions, however, one would expect longer fascicles attaching more rostral segments because they would undergo larger excursion. The uniform fascicle lengths suggest that the psoas is actually designed to move the hip,27 and electromyographic studies confirm that its primary function is hip flexion.30
Extrinsic Muscles Linking Vertebrae or Skull to the Shoulder Girdle or Rib Cage
On the anterior and lateral surface of the neck, the sternocleidomastoid originates from the sternum and medial clavicle to attach on the skull at the mastoid process and superior nuchal line of the occiput (Fig. 3–11). Kamibayashi and Richmond19 divided this muscle into three subvolumes: sternomastoid, cleidomastoid, and cleido-occipital. The fascicles on the superficial surface (sternomastoid and cleido-occipital portions) lie in parallel; however, the cleidomastoid portion on the deep surface, which runs from the clavicle to mastoid process, increases the proportion of muscle fascicles exerting force on the mastoid process (Fig. 3–12).19 Superficial inspection of muscle architecture can neglect the arrangement of these deep fascicles, which would decrease the estimated moment-generating capacity of the sternocleidomastoid in biomechanical models by more than 30%.31 The sternocleidomastoid has moment arms for flexion, contralateral rotation, and lateral bending and has been found to be active during movements in all three of these directions.
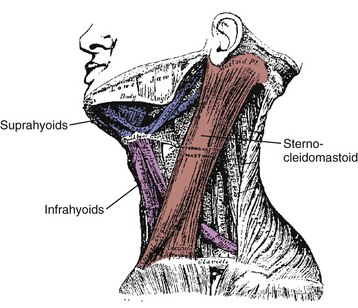
FIGURE 3–11 Lateral view of sternocleidomastoid and hyoid muscles.
(Adapted from Gray H: Gray’s Anatomy. New York, Gramercy Books, 1977.)
Also on the anterior surface of the neck, the infrahyoid muscles (sternohyoid, sternothyroid, thyrohyoid) link the hyoid bone, thyroid cartilage, and sternum, whereas the suprahyoid muscles (digastric, stylohyoid, mylohyoid, and geniohyoid) connect the hyoid bone to the mastoid process and mandible (see Fig. 3–11). The hyoid muscles are generally considered to maneuver the hyoid bone for deglutition and maintaining airway patency, but these muscles could potentially generate a neck flexion moment if the infrahyoid and suprahyoid muscles were activated in concert.
On the posterior surface of the neck, the trapezius is the most superficial muscle (see Fig. 3–6A). It can be divided into three segments: The rostral segment (also called clavotrapezius or trapezius pars descendens) runs from the lateral part of the clavicle to the occiput or ligamentum nuchae, the middle part (acromiotrapezius or pars transversa) runs nearly perpendicular to the midline at the lower cervical and upper thoracic levels from the lateral part of the scapular spine, and the caudal part (spinotrapezius or pars ascendens) attaches to spinous processes of T4 to T12 from the scapula. Its superficial position means that the trapezius has large moment arms for spine and head movements; however, its attachments to the scapula mean that shoulder movements also influence its function. The clavotrapezius (which attaches to the skull) has less than one fifth of the mass of the acromiotrapezius,19 indicating that the trapezius has less moment-generating potential for movements of the skull than generally believed.
Three other muscles connect the scapula to the cervical and thoracic vertebrae. The rhomboideus major and rhomboideus minor run from the medial border of the scapula to the midline at upper thoracic levels. Their major function is retraction of the scapula. The levator scapulae runs from the superior border of the scapula to the transverse processes of upper cervical vertebrae (see Fig. 3–6A). Similar to the trapezius, the functions of these muscles are related to movements of the shoulder.
The scalene muscles (scalenus anterior, medius, and posterior) run from the ribs to transverse processes of cervical vertebrae (see Fig. 3–9). Because of their lateral placement owing to attachments to the ribs, the scalene muscles have substantial moment arms for cervical lateral bending; however, their main function is likely related to respiration. The serratus posterior superior and inferior also attach the vertebral column to the ribs. The serratus posterior superior arises from the lower part of ligamentum nuchae and the spines of the upper thoracic vertebrae and attaches to ribs 2 to 5. The serratus posterior inferior originates from the spines of the lower thoracic and upper lumbar vertebrae and attaches to ribs 9 to 12. These muscles function to elevate and depress the ribs.
The latissimus dorsi arises from the spinous processes of the lower six thoracic and upper two lumbar vertebrae, the thoracolumbar fascia, the iliac crest, and the lower ribs to insert on the humerus. The magnitudes of its potential force and moment on the lumbar spine and sacroiliac joint are small.32 It is generally considered to move the arm, but if the upper limb were fixed, its activity could move the trunk (e.g., as in wheelchair transfers or crutch locomotion).
Implications of Spinal Muscle Anatomy and Architecture for Motor Control
Fascicle Length Changes with Posture
In the cervical spine, many extensor muscles undergo large length changes over the flexion-extension range of motion. A biomechanical model showed that the splenius capitis, semispinalis capitis, semispinalis cervicis, rectus capitis posterior major, and rectus capitis posterior minor all experience fascicle length changes greater than 70% of optimal length over the full range of motion.31 The change in fascicle length depends on the optimal fascicle length of the muscle and the moment arm. The splenius capitis and splenius cervicis have the same optimal fascicle length (see Table 3–3), but the splenius capitis has a much larger moment arm than the splenius cervicis. The splenius capitis undergoes larger fascicle length changes than the splenius cervicis over the same range of motion (Fig. 3–13).
The semispinalis capitis has shorter fascicle lengths, but also a smaller moment arm than the splenius capitis. The semispinalis capitis and splenius capitis experience similar, large fascicle length excursions over the range of flexion-extension motion (see Fig. 3–13). In both muscles, fascicle lengths are extremely short in extended postures; this implies that the central nervous system must compensate for the associated decrease in force-generating potential by increasing activation or recruiting other extensors of the neck.
Moment Arm Changes with Posture
Different parts of a muscle may have different moment arms, and the magnitude (and in some cases, direction) of these moment arms changes with posture. Muscles that cross multiple joints (as most spinal muscles do) may have different mechanical functions at different joints. A biomechanical model of the neck muscles31 showed that the moment arm of the sternocleidomastoid varies dramatically for flexion-extension movements (Fig. 3–14). For motions of the upper cervical joints, the cleido-occipital segment of the sternocleidomastoid actually has an extension moment arm that increases in extended postures (topmost solid line in Fig. 3–14); the other two subvolumes of the sternocleidomastoid (which attach to the mastoid process) have very small moment arms. During flexion of the lower cervical joints, the flexion moment arm of the sternocleidomastoid increases. These results indicate that the function of the sternocleidomastoid depends highly on posture and the joints around which movement occurs. The change in sternocleidomastoid flexion moment arm in the lower cervical region indicates a destabilizing effect because it potentially increases the flexion moment–generating capacity of the muscle in flexed postures.
The same model31 also showed that for axial rotation of the upper cervical region, many muscles have moment arms that vary by 2 to 3 cm but remain in the same direction throughout the range of motion (e.g., sternocleidomastoid, splenius capitis) (Fig. 3–15). For other muscles, the direction of moment arm changes with axial rotation. At the neutral position, the right rectus capitis posterior major has a right rotation moment arm; its magnitude increases in left rotated postures. When the head is rotated to the right, the moment arm decreases in magnitude and eventually changes to a left rotation moment arm. These results indicate that the rectus capitis posterior major has an axial rotation moment arm appropriate to restore the head to neutral posture from the most rotated head positions. The moment arms of other muscles such as the semispinalis capitis and longissimus capitis show the same pattern, although their moment arms are smaller. The implication of these findings is that the moment arm provides a “self-stabilizing” function to assist the central nervous system in maintaining neutrally rotated (i.e., eyes forward) head posture. This function is particularly relevant in the upper cervical region because most axial rotation occurs between C1 and C2.
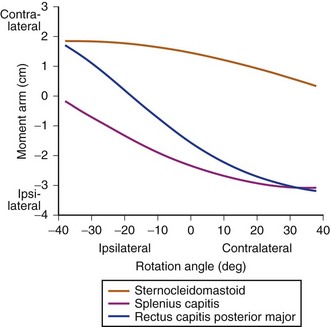
FIGURE 3–15 Axial rotation moment arms for upper cervical region.
(Adapted from Vasavada A, Li S, Delp S: Influence of muscle morphometry and moment arms on the moment-generating capacity of human neck muscles. Spine 23:412-421, 1998.)
In the lumbar spine, posture also changes the mechanical function of erector spine muscles. McGill and colleagues33 measured the fiber angles of longissimus thoracic and iliocostalis lumborum with the lumbar spine in neutral and fully flexed using high-resolution ultrasonography. They found that flexion changes the line of action of these muscles, decreasing their capacity to resist anterior shear forces. This finding is important because anterior shear loads are related to the risk of back injury.34
Electromyography
Knowledge of the complex spinal muscle anatomy is essential for accurate electromyography studies. These studies may be used to detect abnormal muscle activation patterns or to determine input to biomechanical models. MacIntosh and colleagues25 showed the medial fibers of the multifidus (i.e., the fibers immediately lateral to a given spinous process) arise from the spinous process directly above, whereas fibers from higher levels are more lateral. All the fibers of the multifidus arising from a particular vertebra are innervated by the same nerve. This unisegmental innervation has implications for diagnosis of zygapophyseal joint pain related to abnormal activity in the multifidus.
The detailed anatomy of the erector spinae provided by MacIntosh and Bogduk17 also provides important information for electromyography studies. Because thoracic fascicles of the longissimus thoracis and iliocostalis lumborum lie over the lumbar fascicles, electrodes placed at lumbar vertebral levels may not represent activity of fascicles directly attached to lumbar vertebrae. This could result in inaccurate estimation of lumbar joint and tissue loads.
Muscle Injury and Implications for Neck and Back Pain
Muscle pain accompanying eccentric exercise peaks 24 to 48 hours after the exercise bout. Several studies have reported that eccentric exercise results in a significant increase in serum creatine kinase levels 24 to 48 hours after the exercise bout,35,36 and the increase may persist for 3 to 6 days, depending on the precise nature of the exercise. The appearance of creatine kinase in the serum is interpreted as an increased permeability or breakdown of the membrane surrounding the muscle cell.
Based on experimental studies of skeletal muscles directly subjected to eccentric exercise, it is thought that the early events that cause muscle injury are mechanical ones.37,38 During cyclic eccentric exercise of the rabbit tibialis anterior, significant mechanical changes were observed in the first 5 to 7 minutes of exercise.39 Other studies have revealed structural disruption of the cytoskeleton within the fibers at these earlier time periods40,41 that may provide further insights into the damage mechanism.
Animal and human studies have provided evidence for selective damage of fast fiber types after eccentric exercise.42–44 In human studies, this damage was confined to the type 2 muscle fibers in general; in animal studies, damage has been localized further to the type FG (often equated to type 2B) fast fiber subtype. Because FG fibers are the most highly fatigable muscle fibers,45 it has been speculated that the high degree of fatigability of these fibers may predispose them to injury. Several clinical studies have proposed that the fatigability of back muscles may be a predisposing factor to injury. It is difficult to test this idea directly, however, because many other differences between FG fibers and other muscle fibers exist. Further studies are required to elucidate the basis for fiber type–specific injury to skeletal muscle and to document the relationship between muscle injury and back and neck pain.
Implications of Spinal Muscle Anatomy and Architecture for Injury
Muscle Injury Resulting from Eccentric Contraction
As noted earlier, rapid lengthening of muscle is an important mechanism of muscle injury. An example of potential muscle injury secondary to imposed lengthening occurs during whiplash. During the retraction phase of whiplash injury, when the head translates rearward with respect to the torso, the sternocleidomastoid muscle can experience lengthening strains of 5% to 10% while it is active.46,47 During the rebound phase of whiplash injury, when the head translates forward with respect to the torso, the splenius capitis and semispinalis capitis muscles can experience lengthening strains of 10% to 20%. These predictions of muscle strains, based on a biomechanical model that incorporates muscle architecture,31 are above thresholds for strain that causes injury to active-lengthening muscle.37,48,49
Muscles Altering Load Distribution in Other Anatomic Structures
Because muscles are oriented primarily vertically, their activation produces axial compression of the spine. The compressive loads on the discs and facet joints are a function of muscle force, moment arm, and activation. When the detailed anatomy of the lumbar erector spinae was included in a biomechanical model,50 the predicted disc compression and shear loads were reduced compared with a lumped extensor “muscle equivalent” commonly used in many models. This study highlights the importance of accurate representation of muscle anatomy in biomechanical models.
Compressive loads may severely alter tissue loads, particularly if abnormal vertebral kinematics occur. The synovial fold of the facet joint may become impinged during the abnormal kinematics that occur during whiplash.51 Muscles may also contribute to injury by directly loading passive structures. The cervical multifidus has attachments directly to facet capsular ligaments20,52; the combined loading from joint motion and muscle forces may lead to subcatastrophic injuries in facet capsular ligaments. These observations are important because the cervical facet joints and ligaments have been clinically isolated as a source of neck pain.53
Muscle Effects on Spinal Stiffness and Stability
It has long been recognized that muscles are necessary for spinal stability. It is unclear, however, which muscles contribute most to spinal stability; this question has been addressed in several theoretical and experimental studies. Crisco and Panjabi54 examined the role of gross muscle architecture (i.e., the number of joints crossed by a muscle) in lateral stabilization of the lumbar spine using a mathematical model. They calculated minimal muscle stiffness necessary for spinal stability and found that muscles spanning only one vertebral body required the highest stiffness (i.e., activation) for stability, whereas muscles that spanned the largest number of vertebrae were most efficient (required the least activation). Efficient stabilization (less muscle activation) is important because it implies lower disc loads.
Electromyographically driven modeling by Cholewicki and McGill55 suggested that large muscles may provide the bulk of stiffness to the spinal column, as suggested by Crisco and Panjabi,54 but that the activity of short intrinsic muscles was also necessary to maintain stability. Biomechanical models have shown that buckling (loss of stability) can occur from a temporary reduction in activation to one or more intersegmental muscles.55 Presumably, small intrinsic muscles are better suited to stabilize displacements at a single joint with a minimum increase in joint loads at other levels. Similarly, Daru56 and Winters and Peles57 used computer and physical models of the cervical spine to show that activating only large, long muscles resulted in instability, especially around the upright posture. The authors also concluded that activation of deep muscles was necessary for spinal stability. These types of analyses show the importance of gross anatomy and architecture of spinal muscles on spinal stability. Many important questions remain, however, such as the effect of muscle fatigue on spinal stability and the best muscle activation patterns for stability in the prevention and rehabilitation of low back and neck pain.
1 Burke RE, Levine DN, Tsairis P, et al. Physiological types and histochemical profiles in motor units of the cat gastrocnemius. J Physiol (Lond). 1973;234:723-748.
2 Evans WJ, Meredith CN, Cannon JG, et al. Metabolic changes following eccentric exercise in trained and untrained men. J Appl Physiol. 1986;61:1864-1868.
3 Fridén J, Sjöström M, Ekblom B. Myofibrillar damage following intense eccentric exercise in man. Int J Sports Med. 1983;4:170-176.
4 Macintosh JE, Bogduk N. The biomechanics of the lumbar multifidus. Clin Biomech. 1986;1:205-213. MacIntosh JE, Bogduk N: The morphology of the lumbar erector spinae. Spine 12:658-668, 1987; MacIntosh JE, Valencia F, Bogduk N, Munro RR: The morphology of the human lumbar multifidus. Clin Biomech 1:196-204, 1986
5 Warren GW, Hayes D, Lowe DA, et al. Mechanical factors in the initiation of eccentric contraction-induced injury in rat soleus muscle. J Physiol (Lond). 1993;464:457-475.
1 Panjabi M. The stabilizing system of the spine: I. Function, dysfunction, adaptation, and enhancement. J Spinal Disord. 1992;5:383-389.
2 Gans C, Bock WJ. The functional significance of muscle architecture: A theoretical analysis. Adv Anat Embryol Cell Biol. 1965;38:115-142.
3 Gans C, De Vries F. Functional bases of fiber length and angulation in muscle. J Morphol. 1987;192:63-85.
4 Lieber RL. Skeletal Muscle Structure and Function: Implications for Physical Therapy and Sports Medicine. Baltimore: Lippincott, Williams & Wilkins; 2010.
5 Bodine SC, Roy RR, Meadows DA, et al. Architectural, histochemical, and contractile characteristics of a unique biarticular muscle: The cat semitendinosus. J Neurophysiol. 1982;48:192-201.
6 Ounjian M, Roy RR, Eldred E, et al. Physiological and developmental implications of motor unit anatomy. J Neurobiol. 1991;22:547-559.
7 Loeb GE, Pratt CA, Chanaud CM, et al. Distribution and innervation of short, interdigitated muscle fibers in parallel-fibered muscles of the cat hind limb. J Morphol. 1987;1:1-15.
8 Armstrong JB, Rose PK, Vanner S, et al. Compartmentalization of motor units in the cat neck muscle, biventer cervicis. J Neurophysiol. 1988;60:30-45.
9 Richmond FJR, MacGillis DRR, Scott DA. Muscle-fiber compartmentalization in cat splenius muscles. J Neurophysiol. 1985;53:868-885.
10 Burkholder TJ, Lieber RL. Sarcomere length operating range of muscles during movement. J Exp Biol. 2001;204:1529-1536.
11 Lieber RL, Ljung B-O, Fridén J. Intraoperative sarcomere measurements reveal differential musculoskeletal design of long and short wrist extensors. J Exp Biol. 1997;200:19-25.
12 Lieber RL, Loren GJ, Fridén J. In vivo measurement of human wrist extensor muscle sarcomere length changes. J Neurophysiol. 1994;71:874-881.
13 Rome LC, Choi IH, Lutz G, et al. The influence of temperature on muscle function in the fast swimming scup: I. Shortening velocity and muscle recruitment during swimming. J Exp Biol. 1992;163:259-279.
14 Rome LC, Sosnicki AA. Myofilament overlap in swimming carp: II. Sarcomere length changes during swimming. Am J Physiol. 1991;163:281-295.
15 An KN, Takakashi K, Harrington TP, et al. Determination of muscle orientation and moment arms. J Biomech Eng. 1984;106:280-282.
16 Kalimo H, Rantanen J, Viljanen T, et al. Lumbar muscles: Structure and function. Ann Med. 1989;21:353-359.
17 MacIntosh JE, Bogduk N. The morphology of the lumbar erector spinae. Spine. 1987;12:658-668.
18 Delp SL, Suryanarayanan S, Murray WM, et al. Architecture of the rectus abdominis, quadratus lumborum, and erector spinae. J Biomech. 2001;34:371-375.
19 Kamibayashi LK, Richmond FJR. Morphometry of human neck muscles. Spine. 1998;23:1314-1323.
20 Anderson JS, Hsu AW, Vasavada AN. Morphology, architecture, and biomechanics of human cervical multifidus. Spine. 2005;30:86-91.
21 MacIntosh JE, Bodguk N. The biomechanics of the lumbar multifidus. Clin Biomech. 1986;1:205-213.
22 Ward SR, Kim CW, Eng CM, et al. Architectural analysis and intraoperative measurements demonstrate the unique design of the multifidus for lumbar spine stability. J Bone Joint Surg Am. 2009;91:176-185.
23 Donisch EW, Basmajian JV. Electromyography of deep back muscles in man. Am J Anat. 1972;133:25-36.
24 Gray H. Gray’s Anatomy. New York: Gramercy Books; 1977.
25 MacIntosh JE, Valencia F, Bogduk N, et al. The morphology of the human lumbar multifidus. Clin Biomech. 1986;1:196-204.
26 McGill SM, Juker D, Kropf P. Quantitative intramuscular myoelectric activity of quadratus lumborum during a wide variety of tasks. Clin Biomech. 1996;11:170-172.
27 Bogduk N, Pearcy M, Hadfield G. Anatomy and biomechanics of psoas major. Clin Biomech. 1992;7:109-119.
28 McGill SM, Patt N, Norman RW. Measurement of the trunk musculature of active males using CT scan radiography: Implications for force and moment generating capacity about the L4/L5 joint. J Biomech. 1988;21:329-334.
29 Santaguida PL, McGill SM. The psoas major muscle: A three-dimensional geometric study. J Biomech. 1995;28:339-345.
30 Juker D, McGill SM, Kropf P, et al. Quantitative intramuscular myoelectric activity of lumbar portions of psoas and the abdominal wall during a wide variety of tasks. Med Sci Sports Exerc. 1998;30:301-310.
31 Vasavada A, Li S, Delp S. Influence of muscle morphometry and moment arms on the moment-generating capacity of human neck muscles. Spine. 1998;23:412-421.
32 Bogduk N, Johnson G, Spalding D. The morphology and biomechanics of latissimus dorsi. Clin Biomech. 1998;13:377-385.
33 McGill SM, Hughson RL, Parks K. Changes in lumbar lordosis modify the role of the extensor muscles. Clin Biomech. 2000;15:777-780.
34 Norman RW, Wells P, Neumann P, et al. A comparison of peak vs. cumulative physical work exposure risk factors for the reporting of low back pain in the automotive industry. Clin Biomech. 1998;13:561-573.
35 Clarkson PM, Johnson J, Dextradeur D, et al. The relationships among isokinetic endurance, initial strength level, and fiber type. Res Q Exerc Sport. 1982;53:15-19.
36 Evans WJ, Meredith CN, Cannon JG, et al. Metabolic changes following eccentric exercise in trained and untrained men. J Appl Physiol. 1986;61:1864-1868.
37 Lieber RL, Fridén J. Muscle damage is not a function of muscle force but active muscle strain. J Appl Physiol. 1993;74:520-526.
38 Warren GW, Hayes D, Lowe DA, et al. Mechanical factors in the imitation of eccentric contraction-induced injury in rat soleus muscle. J Physiol (Lond). 1993;464:457-475.
39 Lieber RL, McKee-Woodburn T, Fridén J. Muscle damage induced by eccentric contraction of 25% strain. J Appl Physiol. 1991;70:2498-2507.
40 Lieber RL, Schmitz MC, Mishra DK, et al. Contractile and cellular remodeling in rabbit skeletal muscle after cyclic eccentric contractions. J Appl Physiol. 1994;77:1926-1934.
41 Lieber RL, Thornell L-E, Fridén J. Muscle cytoskeletal disruption occurs within the first 15 minutes of cyclic eccentric contraction. J Appl Physiol. 1996;80:278-284.
42 Fridén J. Changes in human skeletal muscle induced by long term eccentric exercise. Cell Tissue Res. 1984;236:365-372.
43 Fridén J, Sjöström M, Ekblom B. Myofibrillar damage following intense eccentric exercise in man. Int J Sports Med. 1983;4:170-176.
44 Lieber RL, Fridén J. Selective damage of fast glycolytic muscle fibers with eccentric contraction of the rabbit tibialis anterior. Acta Physiol Scand. 1988;133:587-588.
45 Burke RE, Levine DN, Tsairis P, et al. Physiological types and histochemical profiles in motor units of the cat gastrocnemius. J Physiol (Lond). 1973;234:723-748.
46 Brault JR, Siegmund GP, Wheeler JB. Cervical muscle response during whiplash: Evidence of a lengthening muscle contraction. Clin Biomech. 2000;15:426-435.
47 Vasavada AN, Brault JR, Siegmund GP. Musculotendon and fascicle strains in anterior and posterior neck muscles during whiplash injury. Spine. 2007;32:756-765.
48 MacPherson PCK, Schork MA, Faulkner JA. Contraction-induced injury in single fiber segments from fast and slow muscles of rats by single stretches. Am J Physiol. 1996;271:C1438-C1446.
49 Patel TJ, Das R, Fridén J, et al. Sarcomere strain and heterogeneity correlate with injury to frog skeletal muscle fiber bundles. J Appl Physiol. 2004;97:1803-1813.
50 McGill SM, Norman RW. Effects of an anatomically detailed erector spinae model of L4/L5 disc compression and shear. J Biomech. 1987;20:591-600.
51 Kaneoka K, Ono K, Inami S, et al. Motion analysis of cervical vertebrae during whiplash loading. Spine. 1999;24:763-770.
52 Winkelstein B, McLendon R, Barbir A, et al. An anatomical investigation of the human cervical facet capsule, quantifying muscle insertion area. J Anat. 2001;198:455-461.
53 Barnsley L, Lord S, Wallis B, et al. The prevalence of chronic cervical zygapophysial joint pain after whiplash. Spine. 1995;20:20-26.
54 Crisco JJ, Panjabi MM. The intersegmental and multisegmental muscles of the lumbar spine: A biomechanical model comparing lateral stabilizing potential. Spine. 1991;16:793-797.
55 Cholewicki J, McGill SM. Mechanical stability of the in vivo lumbar spine: Implications for injury and chronic low back pain. Clin Biomech. 1996;11:1-15.
56 Daru KR. Computer simulation and static analysis of the human head, neck and upper torso MS thesis. Arizona State University. 1989.
57 Winters JM, Peles JD. Neck muscle activity and 3-D head kinematics during quasi-static and dynamic tracking movements. In: Winters JM, Woo SL, editors. Multiple Muscle Systems: Biomechanics and Movement Organization. New York: Springer-Verlag; 1990:461-480.