CHAPTER 2 Applied Anatomy of the Spine
Pars Interarticularis
The pars interarticularis defines the parts of the arch that lie between the superior and inferior articular facets of all subatlantal movable vertebral elements (Fig. 2–1). The term pars interarticularis arose to designate that area of the arch that is most stressed by translational movement between adjacent segments, particularly in the second cervical and fifth lumbar vertebrae, which are susceptible to traumatic and stress fractures in this region (i.e., hangman’s fracture of C21 and isthmic spondylolysis of L5). In sequential alternation with the intervertebral facet joints, it roofs the lateral recesses of the spinal canal and contributes to the dorsal margins of the intervertebral foramina. In the subcervical vertebrae, it also provides the dorsal part of the base of the transverse process.
In the case of the second cervical vertebra (axis) there is a unique anterior relationship of its superior articular facets with the more posteriorly positioned inferior processes that elongates the C2 pars interarticularis. As this offset area receives the greatest leverage between the “cervicocranium” and the lower cervical spine, the indicated line in the illustration in Figure 2–2 shows the common site of mechanical failure in hyperextension injuries to the upper cervical spine.
In the case of the lumbar vertebrae, the pars interarticularis has been subdivided further. McCulloch and Transfelt2 referred to the “lateral buttress,” which they believed offered particular structural support to the intervening structures. They described it as the bony bridge that connects the superolateral edge of the inferior facet to the junction of the transverse process and the pedicle. In a follow-up anatomic study, Weiner and colleagues3 measured the surface area of the lateral buttress in human cadaveric lumbar spines. They found the greatest areas (about 80 mm2) from L1 to L3, whereas area averaged 50 mm2 at L4 and only 15 mm2 at L5. These investigators thought that the broadness of the buttress in the upper lumbar spine can obscure or confuse landmarks for placement of pedicle screws, and its relative thinness (or nonexistence) in the lower lumbar spine can be a predisposing factor to stress fractures or iatrogenic injury to the pars interarticularis.
Regional Characteristics
Although the 24 vertebrae of the presacral spine are divided into three distinct groups (Fig. 2–3), in which the individual members may be recognized by one or two uniquely regional features, there is a gradual craniocaudal progression of morphologic changes. The vertebrae found above and below the point of regional demarcation are transitional and bear some of the characteristics of both areas.
Cervical Vertebrae
Of the seven cervical vertebrae, the first two (Fig. 2–4A to D) and the last require special notation, but the third to the sixth are fairly uniform, and a common description suffices (Fig. 2–4E and F). Because the cervical vertebrae bear the least weight, their bodies are relatively small and thin with respect to the size of the vertebral arch and vertebral foramen. In addition, their diameter is greater transversely than in the anteroposterior direction. The lateral edges of the superior surface of each body are sharply turned upward to form the uncinate processes that are characteristic of the cervical region. The most obvious diagnostic feature of the cervical vertebrae is the transverse foramina that perforate the transverse processes and transmit the vertebral arteries. The anterior part of the transverse processes represents fused costal elements that arise from the sides of the body. The lateral extremities of the transverse processes bear two projections, the anterior and posterior tubercles. The former serve as origins of anterior cervical muscles; the latter provide origins and insertions for posterior cervical muscles. A deep groove between the upper aspects of the tubercles holds the cervical spinal nerves.
The cervical pedicles connect the posterior vertebral arch to the vertebral body. Anatomic studies have shown that the cervical pedicle height ranges from 5.1 to 9.5 mm, and width ranges from 3 to 7.5 mm.4,5 The pedicle is angled medially between 90 and 110 degrees.5
The superior and inferior articular processes appear as obliquely sectioned surfaces of short cylinders of bone that, when united with the adjacent vertebrae, form two osseous shafts posterolateral to the stacked vertebral bodies. The cervical vertebrae present a tripod of flexible columns for the support of the head. As in the upper cervical spine, the combination of the articular processes and the intervening bone is often referred to as the lateral mass in the subaxial region. It is a common site for screw insertion during internal fixation of the cervical spine.6
The laminae are narrow and have a thinner superior edge. At their mid-dorsal junction, they bear a bifid spinous process that receives the insertions of the semispinalis cervicis muscles. The height of the lamina of C4 is 10 to 11 mm, whereas the lamina thickness at C5 is about 2 mm.7 The lamina is thickest at T2, where it measures an average of 5 mm.
Thoracic Vertebrae
All 12 thoracic vertebrae support ribs and have facets for the diarthrodial articulations of these structures. The first and last four have specific peculiarities in the manner of costal articulations, but the second to the eighth are similar (Fig. 2–4G and H).
Because the pedicles arise more superiorly on the dorsum of the body than they do in the cervical region, the inferior vertebral notch forms an even greater contribution to the intervertebral foramen. The pedicle height increases from T1 to T12, but the transverse pedicle width (which is more critical for transpedicular screw containment) does not follow this same craniocaudal pattern.8 Cinotti and colleagues9 found that the pedicles in the T4 to T8 region had the smallest transverse diameter. Scoles and colleagues10 documented similar findings in 50 cadaveric human spines, with the smallest diameters measured at T3 to T6. On average, the transverse pedicle diameter at T3 is 3.4 mm in women and 3.9 mm in men. At T6, it averages 3 mm in women and 3.5 mm in men. At T1, however, the mean diameter is 6.4 mm in women and 7.3 mm in men.
Lumbar Vertebrae
The lumbar vertebrae are the lowest five vertebrae of the presacral column (see Fig. 2–4I and J). All their features are expressed in more massive proportions. They are easily distinguished from other regional elements by their lack of a transverse foramen or costal articular facets. The body is large, having a width greater than its anteroposterior diameter, and is slightly thicker anteriorly than posteriorly. All structures associated with the vertebral arch are blunt and stout. The thick pedicles are widely placed on the dorsolaterosuperior aspects of the body, and with their laminae they enclose a triangular vertebral foramen. Although the inferior vertebral notch is deeper than the superior, both make substantial contributions to the intervertebral foramen. The transverse processes are flat and winglike in the upper three lumbar segments, but in the fifth segment they are thick, rounded stumps. The fourth transverse process is usually the smallest.
Sacral Vertebrae
The sacrum consists of five fused vertebrae that form a single triangular complex of bone that supports the spine and forms the posterior part of the pelvis (Figs. 2–5 and 2–6). It is markedly curved and tilted backward, so that its first element articulates with the fifth lumbar vertebra at a pronounced angle (the sacrovertebral angle).
Arthrology of the Spine
The articulations of the spine include the three major types of joints: synarthroses, diarthroses, and amphiarthroses (Figs. 2-7 to 2-9). The synarthroses are found during development and the first decade of life. The best examples are the neurocentral joints of the immature spine, which are the two unions between the centers of ossification for the two halves of the vertebral arch and that of the centrum. Until they are obliterated during the 2nd decade, they possess a thin plate cartilage between the two apposed bony surfaces. Another example is the early union between the articular processes of the sacral vertebrae, known as ephemeral synchondroses.
Articulations of the Vertebral Arches
This shinglelike arrangement conceals the true length of the ligaments because of the overlapping of the superior lamina. Their morphology is best appreciated from the ventral aspect as in Figure 2–9B. The yellow elastic fibers that give the ligamenta flava their name maintain their elasticity even in embalmed specimens. It has been stated in some texts that the elasticity of the ligamenta flava serves to assist in the maintenance of the erect posture. A more probable reason for this property is simply to keep the ligament taut during extension, where any laxity would permit redundancy and infolding toward the ventrally related nervous structures, as occurs in degenerative lumbar spinal stenosis.
There are two separable layers of the ligamentum flavum, one superficial and one deep, that have distinct attachments to the inferior lamina.11 The superficial component inserts at the classically described location along the posterosuperior aspect of the lamina. The deep component inserts along the anterosuperior surface of the lamina.11 This attachment can have significance during surgical removal of the ligamentum flavum for exposure of the neural elements.
The interspinous ligaments (see Fig. 2–9A) are membranous sets of fibers that connect adjoining spinous processes. They are situated medial to the thin pairs of interspinal muscles that bridge the apices of the spine. The fibers of the ligaments are arranged obliquely as they connect the base of the superior spine with the superior ridge and apex of the next most inferior spinous process. These midline ligaments are found in pairs with a distinct dissectible cleft between them.
The supraspinous ligament (see Fig. 2–9A) is a continuous fibrous cord that runs along the apices of the spinous processes from the seventh cervical to the end of the sacral spinous crest. Similar to the longitudinal ligaments of the vertebra, the more superficial fibers of the ligament extend over several spinal segments, whereas the deeper, shorter fibers bridge only two or three segments. In the cervical region the supraspinous ligament assumes a distinctive character and a specific name, the ligamentum nuchae. This structure is bowstrung across the cervical lordosis from the external occipital protuberance to the spine of the seventh cervical vertebra. Its anterior border forms a sagittal fibrous sheet that divides the posterior nuchal muscles and attaches to the spinous processes of all cervical vertebrae. The ligamentum nuchae contains an abundance of elastic fibers. In quadrupeds, it forms a strong truss that supports the cantilevered position of the head.
Special Articulations
The median atlantoaxial articulation is a pivot (trochoid) joint (Figs. 2–10 and 2–11). The essential features of the articulation are the odontoid process (dens) of the axis and the internal surface of the anterior arch of the atlas. The opposition of the two bones is maintained by the thick, straplike transverse atlantal ligament. The ligament and the arch of the atlas have true synovial cavities intervening between them and the odontoid process. Alar expansions of the transverse ligament attach to tubercles on the lateral rims of the anterior foramen magnum, and a single, unpaired cord, the apical odontoid ligament, attaches the apex of the process to the basion. The entire joint is covered posteriorly by a cranial extension of the posterior longitudinal ligament, which is named tectorial membrane in this region. Because the atlas freely glides over the superior articulating facets of C2, the atlantoaxial pivot is essential for preventing horizontal displacements between C1 and C2. Fracture of the odontoid or, less likely, rupture of the transverse ligament produces a very unstable articulation.
Articulations of the Vertebral Bodies
Intervertebral Disc
In view of the semiliquid nature of the nucleus pulposus and the vacuities that may be shown in the nucleus of aging specimens, von Luschka12 attempted to classify the intervertebral disc as a diarthrosis, in which the vertebral chondral plates were the articular cartilages, the anulus provided the articular capsule, and the fluid and ephemeral spaces within the nucleus corresponded to the synovia and the joint cavity. Although the intervertebral disc forms a joint that should be classified in its own exclusive category because its development, structure, and function are generally different from those of any other joint, it most closely conforms to an amphiarthrosis of the symphysis type.
Anulus Fibrosus
The anulus is a concentric series of fibrous lamellae that encase the nucleus and strongly unite the vertebral bodies (Fig. 2–12). The essential function of the nucleus is to resist and redistribute compressive forces within the spine, whereas one of the major functions of the anulus is to withstand tension, whether the tensile forces be from the horizontal extensions of the compressed nucleus, from the torsional stress of the column, or from the separation of the vertebral bodies on the convex side of a spinal flexure. Without optical aid, simple dissection and discernment reveals how well the anulus is constructed for the performance of this function.
On horizontal section, it is noted that an individual lamella encircling the disc is composed of glistening fibers that run an oblique or spiral course in relation to the axis of the vertebral column. Because the disc presents a kidney-shaped or heart-shaped horizontal section, and the nucleus is displaced posteriorly, these lamellae are thinner and more closely packed between the nucleus and the dorsal aspect of the disc. The bands are stoutest and individually more distinct in the anterior third of the disc, and here when transected they may give the impression that they are of varying composition because every other ring presents a difference in color and elevation with reference to the plane of section. Teasing and inspection at an oblique angle shows in the freed lamellae, however, that this difference is due to an abrupt change in the direction of the fibers of adjacent rings. Previous descriptions of the anulus have claimed that the alternating appearance of the banding is the result of the interposition of a chondrous layer between each fibrous ring.13 In reality, the alternations of glistening white lamellae with translucent rings result from differences in the incidence of light with regard to the direction of the fiber bundles. This repeated reversal of fiber arrangement within the anulus has implications in the biomechanics of the disc, which are discussed later.
The disposition of the lamellae on sagittal section is not consistently vertical. In the regions of the anulus approximating the nucleus pulposus, the first distinct bands curve inward, with their convexity facing the nuclear substance. As one follows the successive layers outward, a true vertical profile is assumed, but as the external laminae of the disc are approached, they may again become bowed, with their convexity facing the periphery of the disc.14,15
The attachment of the anulus to its respective vertebral bodies warrants particular mention. This attachment is best understood when a dried preparation of a thoracic or lumbar vertebra is examined first. In the adult, the articular surface of the body presents two aspects: a concave central depression that is quite porous and an elevated ring of compact bone that appears to be rolled over the edge of the vertebral body. Often a demarcating fissure falsely suggests that the ring is a true epiphysis of the body, but postnatal studies of ossification have indicated that it is a traction apophysis for the attachment of the anulus and associated longitudinal ligaments.16
In life, the depth of the central concavity is filled to the level of the marginal ring by the presence of a cribriform cartilaginous plate. In contrast to other articular surfaces, there is no closing plate of compact osseous material intervening between this cartilage and the cancellous medullary part of the bone. The trabeculations of the spongiosa blend into the internal face of the chondrous plate, whereas fibers from the nucleus and inner lamellae of the anulus penetrate its outer surface. As intimate as this union between the central disc and vertebra may appear, the outer bony ring affords the disc its firmest attachment because the stoutest external lamellar bands of fibers actually penetrate the ring as Sharpey fibers. Scraping the disc to the bone shows the concentric arrangements reflecting the different angles at which the fibers insert (see Fig. 2–12). The fibers of the outermost ring of the anulus have the most extensive range of attachment. They extend beyond the confines of the disc and blend with the vertebral periosteum and the longitudinal ligaments.
Regional Variations of the Disc
The discs in aggregate make up approximately one fourth of the length of the spinal column, exclusive of the sacrum and coccyx. Their degree of contribution is not uniform in the various regions. According to Aeby,17 the discs provide more than one fifth of the length of the cervical spine, approximately one fifth of the length of the thoracic column, and approximately one third of the length of the lumbar region.
The discs are smallest in the cervical spine. Their lateral extent is less than that of the corresponding vertebral body because of the uncinate processes (Fig. 2–13). Here, as in the lumbar region, they are wedge-shaped, the greatest width being anterior, producing lordosis. The thoracic discs are heart-shaped on section, with the nucleus pulposus being more centrally located than in the lumbar region. The thickness and the horizontal dimensions of the thoracic disc increase caudad with the corresponding increase in size of the vertebral bodies. The normal thoracic kyphosis results from a disparity between the anterior and posterior heights of the vertebral bodies because the discs are of uniform thickness. The lumbar discs are reniform and are relatively and absolutely the thickest in the spine. The progressive caudal increase in the degree of lumbar lordosis is due to the equivalent increase in the differential between the anterior and posterior thickness of the disc.
The cervical intervertebral discs have been a source of controversy because of the so-called joints of Luschka, or uncovertebral joints. These articular modifications are found on both sides of the cervical discs as oblique, cleftlike cavities between the superior surfaces of the uncinate processes and the corresponding lateral lips of the interior articular surface of the next superior vertebra. Because they initially appear in the latter part of the first decade and are not universally demonstrable in all cervical spines, or even in all subaxial discs of the same cervical spine, it is preferable to call them “accommodative joints” that have developed in response to the shearing stresses of the torsions of cervical mobility (see Fig. 2–13).
Spinal Ligaments
Anterior Longitudinal Ligament
The anterior longitudinal ligament is a strong band of fibers that extends along the ventral surface of the spine from the skull to the sacrum. It is narrowest and cordlike in the upper cervical region, where it is attached to the atlas and axis and their intervening capsular membranes. It widens as it descends the column to the extent, in the lower lumbar region, of covering most of the anterolateral surfaces of the vertebral bodies and discs before it blends into the presacral fibers. The anterior longitudinal ligament is not uniform in its composition or manner of attachment. Its deepest fibers, which span only one intervertebral level, are covered by an intermediate layer that unites two or three vertebrae and a superficial stratum that may connect four or five levels. Where the ligament is adherent to the anterior surface of the vertebra, it also forms its periosteum. It is most firmly attached to the articular lip at the end of each body. It is most readily elevated at the point of its passage over the midsection of the discs, where it is loosely attached to the connective tissue band that encircles the anulus (Fig. 2–14).
Posterior Longitudinal Ligament
These fibers are most firmly fixed at the margins of their lateral expansions. This produces a central rhomboidal area of loose attachment, or in some cases an actual fascial cleft of equivalent dimensions, on the dorsolateral aspect of the disc. At dissection, this characteristic may be readily shown by inserting a blunt probe beneath the intervertebral part of the longitudinal ligament and exploring the area to define the margins of the space where the fibers are strongly inserted (Fig. 2–15). This situation is particularly pertinent to problems involving dorsal or dorsolateral prolapse of the nucleus pulposus. With a dorsocentral protrusion of a semifluid mass, the strong midline strap of posterior longitudinal fibers tends to restrain the herniation. If an easily dissectible cleft offers a space for lateral expansion, however, the mass can extend to either side, dissecting the loose attachments.
Although not frequently included in anatomic discussions of the spine, an additional structure travels deep to the posterior longitudinal ligament, extending laterally and posteriorly to surround the dura of the cauda equina. It has been termed the peridural membrane, first by Dommissee in 197518 and later by Wiltse.19 The basivertebral veins cross the peridural membrane because it offers no obstruction to vascular communication between the intraosseous vessels of the vertebral body and the epidural space. Its possible clinical significance is that it may provide a containing membrane for herniated discs or hematomas, which may be noted on advanced imaging such as computed tomography (CT) or magnetic resonance imaging (MRI) as a delimiting barrier to the pathology.
Relationships of the Roots of the Spinal Nerves
The nerve root is intimately related to the pedicle of the vertebra. Ugur and colleagues20 found no distance between the upper cervical pedicles and their corresponding nerve roots in 20 cadaveric spines, whereas there was a slight distance in 4 of the 20 specimens in the lower cervical region. For all specimens, the distance from the nerve root to the inferior aspect of the upper pedicle ranged from 1 to 2.5 mm. The distance from the medial aspect of the pedicle to the dural sac ranged from 2.4 to 3.1 mm. A similar relationship between the thoracic nerve roots and pedicle exists.21 The distance from the pedicle to the superior nerve root in the thoracic spine ranges from 1.5 to 6.7 mm, and the distance from the pedicle to the inferior nerve root, 0.8 to 6 mm. Ebraheim and colleagues22 measured these distances in the lumbar spine, finding a mean distance of 1.5 mm from the pedicle to the inferior nerve root, 5.3 mm from the pedicle to the superior nerve root, and 1.5 mm from the medial pedicle wall to the dura.
Of particular interest is the distribution of epidural fat around and within the intervertebral foramen. This fat has a firm character and forms a mechanically supportive “bushing” for structures entering and leaving the spinal canal. A prominent extension of this fat body also follows the inferior and ventral surfaces of each lumbar nerve. It is interposed between the root and the external surfaces of the pedicle and vertebral body that define the inferior part of the intervertebral foramen. Its amelioration of the downward and ventral distraction of the nerve that accompanies the spine and lower limb motions is obvious. Histologically, it is composed of uniform cells that are contained within a fine membrane (perhaps the elusive peridural membrane).23 There is no fibrous tissue in normal epidural fat and only tenuous attachments to the dura.
Intervertebral Foramen
The intervertebral foramen is the aperture that gives exit to the segmental spinal nerves and entrance to the vessels and nerve branches that supply the bone and soft tissues of the vertebral canal. It is superiorly and inferiorly bounded by the respective pedicles of the adjacent vertebrae. Its ventral and dorsal components involve the two major intervertebral articulations. The dorsum of the intervertebral disc, covered by the lateral expansion of the posterior longitudinal ligament, provides a large part of its ventral boundary, whereas the joint capsule of the articular facets and the ligamentum flavum contribute the major parts of its dorsal limitation. Along with the root, the remaining space is filled with loose areolar tissue and fat (Fig. 2–16).
However ample the overall dimensions of the intervertebral foramen may be, its elliptical nature is responsible for many of its relational problems. In the lumbar region, the vertical diameter of the foramen ranges from 12 to 19 mm; this undoubtedly accounts for the fact that a complete collapse of the disc may produce little or no evidence of nerve compression. The sagittal diameter may be only 7 mm, however, making this dimension exquisitely sensitive to changes. Because the diameter of the fourth lumbar nerve can be just slightly less than 7 mm, the tolerance for pathologic alteration of the bony or connective tissue relationships is restricted.24
The existence of additional ligamentous elements in relation to the intervertebral foramen could limit further the space for the exiting spinal nerve. These structures, known as the transforaminal ligaments, are frequently found in the lumbar region.25,26 The transforaminal ligaments are strong, unyielding cords of fibrous tissue that pass anteriorly from various parts of the neural arch to the body of the same or the adjacent vertebra and may be 5 mm wide. Grimes and colleagues27 found these ligaments span from the nerve root itself. These investigators noted four different bands, the most significant of which spread from the nerve root to the anterior aspect of the facet capsule. Other bands spanned from the nerve root to the superior pedicle, the inferior pedicle, and the intervertebral disc anteriorly.
In the cervical spine, the space available for the exiting nerve root may be compromised by structures just lateral to the foramen. In 10 adult human cadaveric specimens, Alleyne and colleagues28 found the dorsal root ganglia of the C3 to C6 spinal nerves to be slightly compressed by the ascending vertebral artery. This compression was most pronounced at the C5 level, which the authors suggested as a possible explanation for the greater susceptibility of this nerve to iatrogenic injury during procedures such as laminoplasty.
Lumbosacral Nerve Root Variations
The most common variation involves atypical origins, or foraminal exits, of individual lumbosacral roots. Although myelographic studies indicated only a 4% incidence of lumbosacral root anomalies, an anatomic study by Kadish and Simmons29 reported an incidence of 14%. The L5-S1 level is the most commonly involved. Observations by these authors provided four types of variations: (1) intradural interconnections between roots at different levels, (2) anomalous levels of origin of nerve roots, (3) extradural connections between roots, and (4) extradural division of nerve roots.
A source of confusing neurologic findings may relate to the variant anatomy of the furcal nerve. The name furcal nerve has been applied to the fourth lumbar nerve because it exhibits a prominent bifurcation to contribute to the lumbar plexus (femoral and obturator nerves) and sacral plexus (lumbosacral trunk). Kikuchi and Hasue30 found that it is often indefinite in its intradural affinities, frequently exhibiting two dorsal root ganglia that have distinct root sources at the conus medullaris. They proposed that when symptoms indicate the involvement of two levels, suspicion should be directed toward four possible causes: (1) two roots compressed by a single lesion, (2) the presence of two lesions, (3) the anomalous emergence of two roots through the same foramen, or (4) the existence of the peculiarly doubled components of the furcal nerve (Fig. 2–17).
Infrequently, variant “fixation” alters the expected sequences of nerve root exit. In a prefixed lumbosacral plexus, the furcal nerve (the division between the lumbar and sacral plexuses) exits through the third lumbar foramen, and the preceding and subsequent nerves exit one vertebral level higher than in the conventional distribution. Conversely, in the postfixed plexus, the furcal nerve exits the L5-S1 foramen, and the lumbosacral nerve sequence is all one level lower than usually described.31
Although Kadish and Simmons29 noted that the existence of anomalous interconnections between nerve root levels dispels any notion of “absolute innervation,” Parke and Watanabe32 showed that there is a consistent system of intersegmental connections between the roots of the lumbosacral nerves. They described an epispinal system of motor axons that courses among the meningeal fibers of the conus medullaris and virtually ensheathes its ventral and lateral funiculi between the L2 and S2 levels. These nerve fibers apparently arise from motor neuron cells of the ventral horn gray matter and join spinal nerve roots caudal to their level of origin. In all the spinal cords studied, many of these axons commingled at the cord surface to form an irregular group of ectopic rootlets that could be visually traced to join conventional spinal nerve roots at one to several segments inferior to their original segmental level (Figs. 2–18 and 2–19). Occasionally, these ventral ectopic rootlets course dorsocaudad to join a dorsal (sensory) nerve root. Although the function and the clinical significance of this epispinal system of axons have yet to be explained, a given segmental level of motor nerve cells may contribute fibers not only to an adjacent segment, but also to nerve roots of multiple inferior levels.
An additional variant aspect of the lumbosacral nerve roots concerns the relative location of their dorsal root ganglia. Almost all anatomic illustrations depict the lumbosacral dorsal root ganglia in an intraforaminal position, the central part of the ganglion lying between the adjacent pedicles. Hasue and colleagues33,34 found, however, that the lumbosacral dorsal root ganglia may also be positioned internal or external to their foramina. They designated the internal positions as subarticular or sublaminar, depending on their relationship to these structures roofing the spinal canal, and found that approximately one third of the L4 and L5 ganglia are in the subarticular position. If the ganglion is subarticular, it is in the lateral recess and subject to the direct consequences of a lateral stenosis.
Innervation of the Spine
Many investigations have attempted to delineate the origins, terminal ramifications, and nerve ending types of the sinuvertebral nerve, often with contradictory results. More comprehensive works15,35–42 have agreed on the general source and composition of this nerve and have described it as variously branching from the distal pole of the dorsal root ganglion, the initial part of the spinal nerve, or the dorsal sections of the rami communicantes. It was recognized that a multiple origin is common, especially in the lumbar region, and small autonomic branches often have a separate course, entering the intervertebral foramen independently. The extent and complexity of the relationships of the sinuvertebral nerve within the spinal canal have engendered much argument, however, particularly concerning the segmental range of the individual nerve ramifications.
In illustrations based on dissections, Bogduk and colleagues35 and Parke43 agreed that each nerve supplies two intervertebral discs via superiorly and inferiorly directed branches—the inferiorly directed branch ramifying over the dorsum of the disc at the level of entry and the longer, superiorly directed branch coursing along the edge of the posterior longitudinal ligament to reach the disc of the next superior level (Fig. 2–20). Dissections identify mainly the larger ramifications. Smaller fibers are usually localized with staining techniques. Conventional methods of staining using silver or lipotrophic stains have given controversial results, however, because of a lack of specificity.
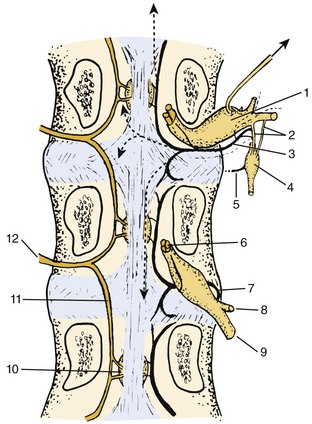
FIGURE 2–20 Schema of major intraspinal distribution of dorsal central branches of segmental vertebromedullary arteries and distribution and source of the sinuvertebral nerves. The pattern of the nerve shown entering the superior foramen is derived from the data provided by Groen and colleagues.44 Dotted lines show a composite of the variant ranges (arrows indicate two or more segments) and ramifications tabulated by these authors. The nerve entering the inferior foramen shows the extent and distribution described in previous reports.
Groen and colleagues,44 using a highly specific acetylcholinesterase staining method on large cleared sections of fetal human spines, resolved many conflicts concerning the ramifications of the nerves supplying spinal structures. They found that, in contrast to most previous reports, the human sinuvertebral nerves were almost exclusively derivatives of the rami communicantes close to their connections with the spinal nerves. These origins were fairly consistent throughout the length of the thoracolumbar sympathetic trunk, but in the cervical region they were also derived from the perivascular plexus of the vertebral artery.
The acetylcholinesterase technique used by Groen and colleagues44 made it possible to delineate details of the plexus of the posterior longitudinal ligament. The work of these authors supports the idea that the posterior longitudinal ligament is highly innervated by an irregular plexiform distribution of fibers that have a greater density in the ligament expansions dorsal to the discs. These authors were able to note the primary direction, length, and “termination area” of the branches of a single segmental sinuvertebral nerve. They classified the variations of individual nerves as follows: (1) ascending one segment, (2) descending one segment, (3) dichotomizing toward one segment caudal and one segment cranial or horizontal, (4) ascending two or more segments, and (5) descending two or more segments (see Fig. 2–20). The existence of the latter two categories, although they are not as common as the others, shows that the sinuvertebral nerve can supply more than two adjacent segmental levels. A basis for the poor pain localization of an offending disc may be related to the generous distribution possible in the individual sinuvertebral nerve. The large totomounts treated with acetylcholinesterase also showed that the patterns of sinuvertebral nerve distribution to the posterior longitudinal ligament did not display significant regional variations apart from an expected pronounced diminution in the plexus density in the immovable lower sacral region.
The posterior longitudinal ligament is highly innervated with complex encapsulated nerve endings and numerous low-myelinated free nerve endings (Fig. 2–21). The lateral expansion of the posterior longitudinal ligament extends through the intervertebral foramen covering all the dorsal and most of the dorsolateral aspects of the disc. The elevation of this thin, highly innervated strap of connective tissue may provide a significant component of the pain manifest in acute disc protrusions.
The probable range of diverse functions of the sinuvertebral nerve may be indicated by the analysis of its cross-sectional composition. Stained preparations taken from a section near the nerve origin show many small myelinated fibers, although some myelin sheaths are greater than 10 µm in diameter.45 Many of the smaller fibers are postganglionic efferents from the thoracolumbar autonomic ganglia that mediate the smooth muscle control of the various vascular elements within the spinal canal, and many of the larger fibers are involved in proprioceptive functions. Concerning the latter, Hirsch and colleagues37,46 found numerous complex encapsulated nerve endings in the posterior longitudinal ligament (see Fig. 2–21B). It is assumed that these may be associated with the larger myelinated fibers whose postganglionic axons enter the cord to mediate postural reflexes because similar fibers in the cervical region of cats have been shown to be important in tonic neck reflexes.47 It seems, however, that the smaller fibers making up the bulk of the sinuvertebral nerve are afferents, associated with simple, nonencapsulated, or “free” nerve endings that are generally regarded as nociceptive (see Fig. 2–21A).
The fact that the sinuvertebral nerve carries pain fibers has been amply shown by clinical and laboratory experimentation. Direct stimulation of tissues known to be served by the nerve elicits back pain in humans. Pedersen and colleagues45 showed that stimulation of these tissues in decerebrate cats resulted in blood pressure and respiratory changes similar to those elicited by noxious stimuli to known pain receptors in other areas of the body.
Disagreement exists over whether the anulus itself is innervated and, if so, how extensively. The classic work of Hirsch and colleagues46 claimed that nerve endings are only in the dorsal aspect of the most superficial layer of the anulus, and these presumably are from branches of the same nerve fibers that innervate the overlying expansions of the posterior longitudinal ligament.
Pedersen and colleagues,45 Stilwell,48 and Parke43 have failed to show nerve endings in the anulus. Because the connective tissue structures intimately related to the disc show a profusion of nerve endings, Parke43 assumed that their disruption could account for discogenic pain. Inappropriate methodology may account for the failure to show intradiscal nerves. Malinsky,40 Bogduk and colleagues,35,36 and Yoshizawa and colleagues49 published accounts showing nerve fibers in the outer lamina of the anulus. This work has now been supported by the highly specific acetylcholinesterase method of Groen and colleagues.44
Most descriptions of the sinuvertebral nerve indicate that the major meningeal fibers to the spinal dura are distributed to its ventral surface.50 The median dorsal dural surface has been regarded as virtually free of nerve fibers, a convenience that permits its painless penetration during needle puncture. Although Cyriax51 claimed that irritation of the ventral dura during protrusion of the nucleus may contribute to discogenic pain, a sufficient distortion of the nerve fibers on the movable or unattached dura does not seem likely. The coiled configuration of these dural contributions of the sinuvertebral nerve, noted by Groen and colleagues,52 may indicate a compensation to permit a degree of dural movement without placing traction on these nerves.
Parke and Watanabe53 observed that the ventral lower lumbar dura is often fixed to the ventral canal surface by numerous connective tissue fibers, most firmly fixed at the margins of the lower lumbar discs. These apparently acquired adhesions are not to be confused with the ligaments of Hofmann, which are normal straps of tissue connecting the dura to the ventral canal surface that have been obliquely positioned by the developmental cranial traction of the dura and its contents. This observation has been supported by a series of dissections by Blikra,54 who was seeking a rationale for lower lumbar intradural disc protrusions. His analysis showed that in some cases the dura may be sufficiently fixed to the ventral surface of the canal, particularly at the L4-5 level, for protruding nucleus material to rupture the ventral dura. Parke and Watanabe,53 by microscopic analysis of sections of the dura that had been forcibly freed from these adhesions overlying the fourth or fifth lumbar disc, showed disruption of the nerve fibers bound in the adhesion. In the numerous cases in which such adhesions are present, the forceful elevation of the dura by a disc protrusion may provide an adjunctive source of the discogenic pain.
Spinal Motion Segment
The inclusion of all articular tissue, the overlying spinal muscles, and the segmental contents of the vertebral canal and intervertebral foramen into a single functional and anatomic unit was first suggested by Junghanns.55,56 Originally called the “motor” segment, this unit represents a useful concept that stresses the developmental and topographic interdependence between the fibrous structures that surround the intervertebral foramen and the functioning of the structures that pass through it. Although the 23 or 24 individual motion segments must be considered in relation to the spinal column as a whole, no congenital or acquired disorder of a single major component of a unit can exist without affecting first the functions of the other components of the same unit and then the functions of other levels of the spine.
Although Junghanns55 defined the unit primarily in terms of the movable structures making up the intervertebral articulations, a logical, if not necessary, extension of the motion segment concept should include some aspect of the vertebral elements. DePalma and Rothman57 included both adjacent vertebrae in their illustration of the unit, but one of us believed that the unit concept would be improved by incorporating only the opposing superior and inferior halves of each vertebra, eliminating redundancy (see Fig. 2–16). In visualizing the motion segment unit as a musculoskeletal complex surrounding a corresponding level of nervous structures, it must be realized that the intervertebral disc and the facets are but two of the articulations involved. The interosseous fibrous connections that include the interspinous, intertransverse, costovertebral, and longitudinal ligaments and the ligamentum flavum are varieties of syndesmoses.
Nutrition of the Intervertebral Disc
Most descriptive accounts of the intervertebral disc dismiss the subject of its vascular nutrition with a brief mention of the general agreement that the normal adult disc is avascular. The demonstrable truth of this statement may give the impression that the substance of the disc is inert biologically. Experimental evidence has indicated that the normal disc tissue is quite vital and has a demonstrable rate of metabolic turnover.58,59 In contrast to the nonvascular cartilage in the diarthroses, the cellular elements of the disc cannot receive the blood-borne nutrients through the mediation of the synovial fluid but must rely on a diffusional system with the vessels that lie adjacent to the disc.
The qualitative and quantitative aspects of the diffusional nutrition of the disc have been studied.59–62 The peripheral vascular plexus of the anulus and the vessels adjacent to the hyaline cartilage of the bone-disc interface provide the two sources for the diffusion of metabolites into the disc. Although the interface shows an average permeability of 40%, there is a decreasing centrifugal gradient that starts with an 80% permeability at the center. Because diffusion is the major mechanism that carries small solutes through the disc matrix, the two main parameters affecting this flow are the partition coefficient, which defines the equilibrium between the solutes within the plasma and the solutes within the disc, and the diffusion coefficient, which characterizes the solute mobility.
Blood Supply of the Vertebral Column
The descriptions and terminology of the nutritional vessels of the vertebrae vary considerably in anatomy texts. In general, the texts illustrate and discuss the vascularity of a typical thoracic or lumbar vertebra, with a lack of agreement on such basic issues as to whether the vertebral body does63 or does not64 receive an anterior supply. In addition, discussions of the vascularization of the atypical (craniocervical, cervical, and sacral) vertebral regions are either superficial or entirely lacking. Much of the information presented here is the result of a de novo investigation by the senior author (W.W.P.) and his colleagues, and the terminology ascribed to the vessels is derived from a selection of what seem to be the most descriptive names previously used in other reports and the senior author’s reference.65
This general pattern of the vasculature is best shown in the area between the second thoracic and fifth lumbar vertebrae, where the segments are associated with paired arteries that arise directly from the aorta (Fig. 2–22). Typically, each segmental artery leaves the posterior surface of the aorta and follows a dorsolateral course around the middle of the vertebral body. Near the transverse processes, it divides into a lateral (intercostal or lumbar) and a dorsal branch. The dorsal branch runs lateral to the intervertebral foramen and the articular processes as it continues backward between the transverse processes eventually to reach the spinal muscles. Because the segmental artery is closely applied to the anterolateral surface of the body, its first spinal derivatives are two or more anterior central branches that directly penetrate the cortical bone of the body and that may be traced radiologically into the spongiosa (Figs. 2–23 and 2–24). The same region of the segmental artery also supplies longitudinal arteries to the anterior longitudinal ligament (Fig. 2–25).
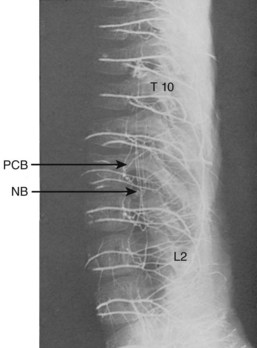
FIGURE 2–25 Lateral view of lumbar vertebra shown in Figure 2–24. Longitudinal anastomoses of posterior central branches (pcb) can be appreciated, and the disposition of neural branches (nb) is clarified. The lumbar arteries also supply small longitudinal branches to the anterior longitudinal ligament.
Coursing in the same plane as the posterior longitudinal ligament, these branches vascularize the ligament and the related dura before entering the large concavity in the central dorsal surface of the vertebral body. The dorsum of each vertebral body is supplied by four arteries derived from two intervertebral levels. As these vessels tend to converge toward the dorsal central concavity, where they are cross-connected with their bilateral counterparts, their connections with other vertebral levels give the appearance of a series of rhomboid anastomotic loops (Fig. 2–26) that illustrate the extent of collateral supply to a single vertebra.
The prelaminar branch of the spinal artery follows the inner surface of the vertebral arch, giving fine penetrating nutrient branches to the laminae and ligamenta flava, while also supplying the regional epidural and dorsal tissue. The neural branches that enter the intervertebral foramen with the above-described vessels supply the pia-arachnoid complex and the spinal cord itself. In the fetus and the adult, the neural or radicular branches are not segmentally uniform in their size or occurrence. Although all spinal nerves receive fine twigs to their ganglia and roots, the major contributions to the cord are found at irregular intervals. Several larger radicular arteries may be discerned in the cervical and upper thoracic regions, but the largest, the arteria radicularis magna (artery of Adamkiewicz66), is an asymmetrical contribution from one of the upper lumbar, or lower thoracic, segmental arteries. It travels obliquely upward with a ventral spinal root to join the anterior spinal artery in the region of the conus medullaris. Radicular contributions to the dorsal spinal plexus may usually be distinguished by their more tortuous course (see Figs. 2–25 and 2–26).
Regional Variations in Spinal Vasculature
Only vertebrae that are related to the aorta have access to direct segmental branches. The cervical, upper thoracic, and sacral regions have different patterns in their segmental supply that affect to various extents the arrangements of the finer vessels. In an arteriogram of the entire fetal spine (see Fig. 2–22), it can be seen that the greater part of the cervical region is supplied by the vertebral arteries and the deep cervical arteries. An intermediate area that usually includes the lower two cervical and upper two thoracic vertebrae is supplied by costocervical branches of the subclavian artery that are of variable pattern and often bilaterally dissimilar. From T2 to L3, the typical segmental arrangement prevails, but in the sacral area lateral sacral branches of the hypogastric artery and middle sacral branches assume the function of supporting the nutritional vasculature to the vertebral elements.
Cervical Region
The general patterns of the arterial supply with respect to the typical cervical vertebrae are schematically represented in Figures 2–27A and 2–28.67 The vertebral arteries represent a lateral longitudinal fusion of the original segmental vessels and provide a ventrally coursing anterior central artery and a medially directed posterior central artery to each subaxial vertebral element. The anterior spinal plexus is best developed in the cervical region, where it exhibits a rectangular mesh of vessels in which the transverse members (anterior central arteries) run along the upper ventral edges of their respective intervertebral discs. The conspicuousness of this plexus reflects the fact that it also serves the cervical prevertebral musculature. The thyrocervical and costocervical trunks assist in the lower cervical region, and the upper cervical part of the plexus receives contributions from the ascending pharyngeal arteries (Fig. 2–29).
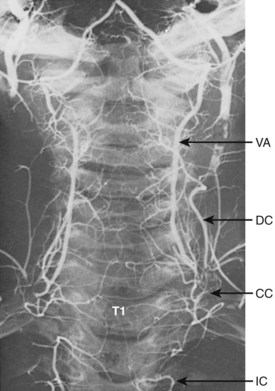
FIGURE 2–29 Arteriogram of cervical and upper thoracic regions of the 6-year-old spine seen in Figures 2–23 and 2–24. The vertebral artery (va) and deep cervical branch (dc) of the costocervical trunk (cc) supply segmental branches to each vertebra. The costocervical artery also typically supplies T1 and T2, but in this case T2 receives a high intercostal (ic) branch on the left side.
Atlantoaxial Complex
In light of the foregoing facts, it was not unexpected that the investigations of Schiff and Parke68 revealed that the odontoid process was supplied primarily by pairs of anterior and posterior central branches that coursed upward from the surfaces of the body of the axis and were derived from the vertebral arteries at the level of the foramen of the third cervical nerve. The posterior ascending arteries are the larger members of these two sets of vessels and usually arise independently from the posteromedial sides of their respective vertebral arteries. The individual artery enters the vertebral canal through the foramen between the second and third vertebrae and trifurcates on the dorsum of the axis body. The typical posterior central perforators course medially passing deep to the posterior longitudinal ligament (called the tectorial membrane in the craniocervical region) to penetrate into the spongiosa of the axis. A small descending branch anastomoses distally with vessels of the next lower segment.
The major part of the posterior ascending artery crosses the dorsal surface of the transverse ligament of the atlas about 1.5 mm lateral to the neck of the odontoid process (see Fig. 2–27). Dorsal to the alar ligament, it sends an anterior anastomotic branch over the cranial edge of this ligament to form collateral connections with the anterior ascending artery. The posterior ascending artery continues on a medial course to meet its opposite counterpart and forms the apical arcade that arches over the apex of the odontoid process.
Collateral vessels pass over and under the anterior arch of the atlas to anastomose with the apical arcade and ascending arteries.68 These are derived from some component of the external carotid system. These vessels are branches of the ascending pharyngeal artery, which has a nearly ubiquitous distribution in the upper pharyngeal region and sends a branch along the inner aspect of the carotid sheath that, on reaching the base of the skull, becomes recurrent and descends deep to the prevertebral fascia to supply the upper prevertebral cervical muscles and anastomose with the anterior spinal plexus. Numerous small-bore vessels that descend from the rim of the foramen magnum to anastomose with the apical arcade are derivatives of a meningeal branch of the occipital artery that enters the skull through the hypoglossal canal (see Fig. 2–27). Its descending branches supply the periforaminal dura, the tectorial membrane and alar and apical ligaments, and the fine anastomoses to the arcade.
Sacroiliolumbar Arterial System
With the increasing use of percutaneous approaches to the lower lumbar discs, this infra-aortic system of vessels has assumed some surgical significance, particularly because, in contrast to the conventional segmental supply to the more superior vertebrae, its major components are longitudinally related to the dorsolateral surfaces of the discs most frequently involved in these procedures.69
Fourth Lumbar Arteries
As depicted in Figures 2–30 and 2–31, the distribution of the major ramifications is similar to that of the thoracic segmental vessels, with the exception of additional branches that supply the psoas and quadratus lumborum muscles. The lateral muscular branch (equivalent of the thoracic intercostals) may be quite large at the fourth lumbar level, where, in contrast to the other lumbar laterals, it passes anterior, rather than posterior, to the quadratus lumborum. It then continues to supply the lower posterolateral abdominal wall as it courses superior to the crest of the ilium. As can be seen in Figure 2–30, it may be equivalent in size to the iliac branch of the iliolumbar artery. Its position superior to the crest indicates that it is more likely to be encountered by percutaneous instrumentation than the latter vessel.
The dorsal musculocutaneous branch of the fourth lumbar artery is equivalent in distribution to other thoracolumbar segmental arteries. It usually has a medial branch that supplies the external aspects of the facet joints and neural arch components and the transversospinal group of muscles and a lateral branch to the transversocostal group of the erector spinae. The vertebromedullary (spinal) branches of the fourth lumbar artery are also similar to those of other segmental arteries (see Fig. 2–24). They are a group of vessels of variable caliber that may generally be sorted into three divisions: (1) the ventral periosteal and osseous branches that supply the posterior longitudinal ligament, the periosteum, and the cancellous bone of the vertebral body; (2) the radiculomedullary division that provides the irregularly located medullary arteries of the cord and the constant distal radicular arteries to all the roots; and (3) the dorsal division that supplies fine articular branches to the deep aspects of the facet joints and the periosteum of the deep surfaces of the laminae and their associated ligaments. The first two divisions usually originate from a common branch of the segmental artery and enter the intervertebral foramen just rostral to their respective vertebral pedicle and ventral to the dorsal root ganglion, whereas the dorsal division arises from the musculocutaneous branch of the segmental artery and enters the foramen dorsal to the nerve components. All the vertebromedullary branches may provide fine branches to the spinal dura.
A major peculiarity of the fourth lumbar artery is its proclivity toward providing a relatively large, caudally directed intersegmental branch that arises near the level of the intervertebral foramen and becomes reciprocally involved with the lumbar branch of the iliolumbar artery. When this latter vessel is small or absent, the descending branch of the fourth lumbar artery may be sufficiently large to provide the predominant nutritional system to two vertebral segments caudad to its origin (see Figs. 2–30 and 2–31).
Iliolumbar Artery
The lumbar artery ascends posterolateral to the L5-S1 disc, still between the obturator nerve and the lumbosacral trunk, to provide the vertebromedullary vessels to the L5-S1 intervertebral foramen (Fig. 2–32; see Figs. 2–30 and 2–31). In most cases, a branch of this vessel continues rostrally to anastomose with the descending branch of the fourth lumbar artery. The lumbar branch of the iliolumbar artery provides regional branches to the psoas and quadratus lumborum muscles.
Sacral Arteries
Middle Sacral Artery
The middle sacral artery is an unpaired vessel that is the last branch of the aorta, usually derived from its dorsal median surface just above the carina of the bifurcation (Fig. 2–33; see Fig. 2–30). It descends down the ventral surface of the anterior longitudinal ligament over the fourth and fifth lumbar bodies and down the ventral sacrum to terminate at the sacrococcygeal junction in a vascular glomus (sacrococcygeal body) in tail-less mammals or continues ventral to the coccygeal (caudal) vertebrae in tailed mammals as the caudal artery. In humans, this is a variable vessel, being totally absent in some cases or replaced by a branch of one of the lateral sacral arteries. Where it is a significant component of the sacroiliolumbar system, its first lateral branches on the ventral surface of the fifth lumbar body may entirely replace this segment’s contributions from the iliolumbar or fourth lumbar vessels and provide its osseous, muscular, and vertebromedullary requirements.
Functional Significance
The sacroiliolumbar system, despite its complexity and seemingly endless combinations of reciprocal substitutions, supplies the lower lumbosacral elements of the spine and the inferior half of the lumbosacral spinal nerve roots (cauda equina) and the back musculature inferior to the L4 level. It is also a major contributor to the vasa nervorum of the lumbosacral plexus. The distal radicular arteries define the positions of the lumbosacral roots (see Fig. 2–25). Although significant medullary branches to the spinal cord are seldom found below L4, they do occur, and from the preceding descriptions it is obvious why the ligation of both internal iliac arteries during radical cystoprostatectomy can result in spinal cord ischemia.70
Venous System of the Vertebral Column
The internal venous plexus is of more functional and anatomic interest. This plexus is essentially a series of irregular, valveless epidural sinuses that extend from the coccyx to the foramen magnum. Its channels are embedded in the epidural fat and are supported by a network of collagenous fibers, but their walls are so thin that their extent or configuration cannot be discerned by gross dissection. This latter property may account for the fact that the epidural venous sinuses have been periodically “rediscovered.” The epidural vertebral veins were known to Vesalius and his contemporaries and were described and illustrated in the first part of the 19th century by Breschet.71 Batson,72 Clemens,73 and others made the functional and pathologic significance of these vessels apparent (Fig. 2–34).
The plexus does not entwine the dura in a completely haphazard fashion but is arranged in a series of cross-connected expansions that produce anterior and posterior ladderlike configurations up the vertebral canal. The main anterior components of the epidural plexus consist of two continuous channels that course along the posterior surface of the vertebral bodies just medial to the pedicles. These channels expand medially to create cross anastomoses over the central dorsal area of each vertebral body and are thinnest where they overlie the intervertebral discs. When injected with a contrast medium, the main channels may appear as a segmental chain of rhomboid beads. Chaynes and colleagues74 studied the internal venous plexus using silicon injection techniques. They found that anterior longitudinal veins were located in a “dehiscence” within the periosteum along the lateral aspect of the spinal canal and that veins of each side communicated with each other through a retrocorporeal vein. In the cervical spine, the retrocorporeal vein was found deep to the posterior longitudinal ligament, whereas it was superficial to the ligament in the thoracic and lumbar regions.
The major external connections of the epidural plexus consist of the veins that pass through the intervertebral foramen and eventually empty into the segmentally available intercostal or lumbar veins (Fig. 2–35). Because these sinuses are valveless, one cannot refer accurately to directions of drainage and flow. The greatest functional significance of these vessels lies in their ability to pass blood in any direction according to the constantly shifting intra-abdominal and intrathoracic pressures. Breschet71 surmised that the epidural plexus served as a collateral route for the valveless caval and azygos systems. This ability has been shown by experimental ligation of either the superior vena cava or the inferior vena cava. In addition, the Queckenstedt maneuver, which tests the patency of the spinal subarachnoid space by compressing the jugular or intra-abdominal veins, causes an increase in cerebrospinal fluid pressure through dural compression from the expansion of the collaterally loaded epidural plexus.
The plexus is evidently capable of passing large quantities of blood without developing varices. Clemens claimed that this feature was due to the intricate network of collagenous fibers that supports the thin walls of the sinuses. Also, passive congestion of the spinal cord is prevented by minute valves in the radicular branches draining the spinal cord.73 This latter fact is anatomically unique because valves exist nowhere else in the venous channels associated with the central nervous system. An ancillary function of the epidural plexus may be to act in a mechanical capacity as a hydraulic shock-absorbing sheath that helps buffer the spinal cord during movements of the vertebral column, similar to the epidural fat.
The vertebral sinuses are largest in the suboccipital and upper cervical region. Here they also receive numerous nerve endings from the sinuvertebral nerves and are associated with glomerular arteriovenous anastomoses, which suggests a possible baroceptive function.75 The patency of these anastomoses is most easily shown in the fetus, in which arterial injections of a contrast medium may also fill the upper cervical epidural sinuses. Similarly, the coccygeal bodies of the same specimen pass the arterial injection directly into the epidural veins of the lower sacral region.
The detrimental aspects of the vertebral epidural veins have been well stated by Batson.72 Retrograde flow from venous connections to the lower pelvic organs provides an obvious route of metastasis for pelvic neoplasms to the spine itself and to the regions of the trunk associated with valveless connections to the plexus. Batson72 claimed that direct metastatic transfer can occur between the pelvic organs and the brain via the vertebral epidural route.
Another extraspinal-intraspinal venous connection implicated in the transfer of pathologic processes involves the pharyngovertebral veins.76 These vessels constitute a system that drains the superior posterolateral regions of the nasopharynx and coalesces into two to several veins that penetrate the anterior atlanto-occipital membrane to discharge into the venous complex surrounding the median and lateral atlantoaxial joints. Because posterior pharyngeal infections have been linked with the atlantoaxial rotatory subluxations characteristic of Grisel syndrome,77 it is believed that the pharyngovertebral veins are instrumental in transporting infectious processes that may produce a hyperemic relaxation of the atlantoaxial ligaments. The existence of this venous system also explains the ease in transfer of superior pharyngeal metastatic processes to the upper cervical epidural veins.
Blood Supply of the Spinal Cord
Throughout the length of the spinal cord, a system of three longitudinal vessels receives blood from the irregularly located medullary branches of the segmental spinal arteries and distributes it to the substance of the cord. This system consists of the single median ventral anterior spinal artery and two smaller dorsolateral spinal arteries (Fig. 2–36).
Anterior Spinal Artery
Despite its great functional significance, the anterior spinal artery remains one of the more inaccurately described and inadequately understood blood vessels. Derived from the fusion of bilateral pairs of ascending and descending anastomotic branches of the original segmental arteries of the developing spinal cord,78 this median ventral pial vessel supplies approximately 80% of the intrinsic spinal cord vasculature. It is usually depicted in texts as a single continuous artery of nearly uniform caliber that extends from the medulla oblongata to the conus. The anterior spinal artery is actually a longitudinal series of functionally independent vessels that may show wide luminal variations and anatomic discontinuities.78–80
Although the investigations of Crock and Yoshizawa65 have tended to minimize the significance of predominant regional feeders, many functionally oriented reports have claimed that the cord has three major arterial domains along its vertical axis: (1) the cervicothoracic region (C1-T3), (2) the mid-thoracic region (T3-8), and (3) the thoracolumbar (including sacral cord) region (T8-conus). The reports have also claimed that these areas have little anastomotic exchange between their junctions (Fig. 2–37).
Brewer and colleagues79 and Lazorthes and associates80 maintained that a series of human anterior spinal arteries consistently show interruptions, or critically narrow zones, in the mid-thoracic region, and these influence the potential collateral blood flow along the longitudinal axis of the cord. It is not only the observed size of the vessel that is of physiologic significance, however. The existence of a marked autoregulatory control of the intrinsic spinal cord blood flow has been independently shown in many mammalian species.24,81 Microscopic investigation82 of sections of the descending and ascending contributions of the arteria medullaris magna (artery of Adamkiewicz, also known as the arteria radicularis magna) to the anterior spinal artery showed that these arteries, in addition to their well-developed circumferential muscle of the tunica media, also possess a layer of predominantly longitudinal intimal musculature. Located between the internal elastic lamina and the endothelium, this layer ranges in thickness from one fifth to one half of the tunica media (Fig. 2–38).
In following a series of cranial to caudal sections of the thoracolumbar anterior spinal artery, it was noted that the intimal muscle layer did not extend into any of its branches. At the mouth of the central (sulcal) arteries, which are the largest anterior spinal artery derivatives, the intimal musculature stops abruptly, often forming a liplike projection over the opening of the branch vessel, but no intimal muscle fibers extend into the central arteries. A sphincter-like thickening of the central artery tunica media, seen at the ostium of the vessels, indicates that this muscle layer has a greater contractile influence at this point (see Fig. 2–38). The intimal musculature, in addition to enhancing the luminal control of the anterior spinal artery, also is involved in controlling the blood flow into the central arteries. Where the intimal layer shows the liplike projections, successive serial sections indicate that contraction of the longitudinally disposed intimal muscle fibers forms an ellipsoidal buttonhole-shaped orifice whose long axis is parallel to that of the fiber orientation. Such an arrangement permits exquisite muscular control of the blood flow from the anterior spinal artery to its central artery branches.
In addition to the fairly uniform layer of the intimal musculature throughout the walls of the examined sections of the thoracolumbar anterior spinal artery, serial sections cut through the arch-shaped junction of the arteria medullaris magna and the descending anterior spinal artery branches show that this intimal layer, in most cases, is organized into prominent intimal cushions. These muscular thickenings are erratically distributed along the lumen of the hairpin-shaped arterial arch and the initial segment of the ascending branch of the anterior spinal artery (Figs. 2–39 and 2–40). This latter location is of considerable interest because its prominent cushions, with reinforced thickenings of the underlying tunica media, could exert considerable influence over the quantity of blood flow between the thoracolumbar and mid-thoracic vascular domains. This intimal control system, when coupled with the intramedullary arteriovenous anastomoses (described in a subsequent section on intrinsic vascularity), provides an anatomic basis for the dramatic range of spinal cord blood flow autoregulation. The presence of the intimal cushions explains the often-noted failure of the arteria medullaris magna to supply adequately the mid-thoracic cord region above the arteria medullaris magna–anterior spinal artery junction during aortic cross clamping.
The ventral position of the anterior spinal artery and its nutritional importance may have consequence in spinal stenosis. Particularly in the lower cervical region, its compression by dorsal osteophytes and cartilaginous protrusions related to cervical disc degeneration may lead to the neurologically disastrous anterior spinal artery syndrome.83 The medullary feeder arteries that supply the anterior spinal artery may arise from any spinal segmental artery. Studies by Dommissee84 showed, however, that there are statistical preferences for certain segmental levels. There are usually three anterior medullary arteries for the cervical region, one or two for the thoracic region, and a conspicuous medullary vessel (the arteria medullaris magna) for the lumbosacral cord region. The levels of origin for all these vessels center around certain “average” locations in each region. The anterior spinal artery is usually of greatest caliber in the lumbosacral part of the cord, where it supplies the considerable tissue mass of the proximal cauda equina in addition to the lumbosacral cord intumescence.
Lateral Spinal Arteries of the Cervical Cord
Although these vessels were observed in the later 19th century, they were usually regarded as variants, and their functional significance was not appreciated. Lasjaunias and colleagues85 compiled an extensive report on the variations and selective angiography of these important vessels.
Intrinsic Vascularity of the Spinal Cord
The tissues of the spinal cord are supplied by two systems of vessels that enter its substance. The first is a centripetal arrangement of arteries that supplies the superficial tracts of the ventral and lateral funiculi, all of the dorsal funiculus, and the extremities of the dorsal horns. They are radially penetrating branches of the vasa corona and the dorsolateral spinal arteries, which serve only a little more than one fourth of the cord. The greater part of the cord and almost all of its gray matter is supplied by a second centrifugal system of vessels derived from the sulcal (or central) arteries.86 These arteries are a repetitive series of branches derived from the dorsal aspect of the anterior spinal artery that penetrate the depths of the anterior median fissure. In the mid-sagittal plane, they form a close palisade of vessels that occur with a frequency of 3 to 8 arteries per 1 cm in the cervical region and 2 to 6 per 1 cm in the thoracic cord; they are densest in the lumbar region, where they number 5 to 12 per 1 cm of the anterior spinal artery. The average diameters of the sulcal arteries are greater in the cervical (0.21 mm) and lumbosacral (0.23 mm) regions than in the thoracic cord (0.14 mm).87
As these vessels approach the anterior commissure, most turn to either the right or the left and supply only the corresponding side of the cord.8,63,88,89 This unilateral proclivity reflects their origins in the early embryonic stages when the anterior spinal arteries first condensed from a primitive plexus as a symmetrical pair of longitudinal vessels, each supplying its respective half of the cord. In subsequent development, these two vessels fused in the midline to form the definitive single median anterior spinal artery, but their sulcal branches retained their original unilateral affinities. Bilateral distributions occur in 9%, 7%, and 14% of the cervical, thoracic, and lumbar vessels.87,90
The greater metabolic requirements of the spinal gray matter, in contrast to the funicular tissue, are dramatically reflected in their relative capillary densities. Quantification of the microvascularity in the spinal cord has shown that the capillary density of the gray matter is four to five times as great as the white matter.91 The capillary distribution within the gray matter is not homogeneous, however, and varies with the regional concentrations of the nuclei. The nuclei of the dorsal horn are fairly uniformly distributed. The ventral horn shows segmental nuclear clusters, which display distinct nerve cell groups.
As noted by Feeney and Watterson,92 the capillary densities of the white and gray matter of the central nervous system are established at a level that is minimally requisite for the metabolic needs of the given tissue. This situation is in contrast to most other body tissues, which have a capillary “reserve” and normally function with only part of their capillary channels open, varying their intrinsic vascular resistance by dilation of the accessory channels. Nevertheless, despite the lack of this method of control, the spinal cord exhibits a remarkable range of blood flow autoregulation.1,24,93 The intrinsic cord vasculature maintains a constant blood flow throughout a wide range of systemic blood pressure alterations, although each animal species has a definite upper and lower limit to the systemic blood pressure at which the regulation decompensates. Because transection of the upper cervical cord has no effect on this autoregulatory capacity, it may be assumed that this reflex is local and independent of autonomic nerve control.
Numerous third-order branches of the sulcal arteries communicate directly with veins through convoluted anastomoses. These vascular structures are located primarily in the area that divides the ventral two thirds of the dorsal horn from the dorsal one third and in the more central regions of the ventral horn. They show a paucity of contractile elements and instead exhibit an “epithelioid” type of media that seems capable of swelling and diminishing its thickness. Because this action could rapidly control the caliber of the anastomotic lumina in immediate response to local metabolic changes, these anastomotic convolutions may be the site of the reflex adjustment in the flow resistance of the spinal cord vasculature.94
Perhaps the most essential part of knowledge of the vascular supply of the spinal cord is awareness of the ranges of individual variability. The numerous successful surgical cases in which the arteria medullaris magna has been inadvertently interrupted without producing a disastrous spinal cord ischemia give the impression that an adequate collateral vascularity may protect the cord in most individuals when a single major artery is compromised. In procedures involving the interruption of blood flow in numerous consecutive segmental branches of the aorta, such as aortic cross clamping for abdominal vascular surgery, the maintenance of adequate spinal cord blood flow, particularly in the thoracic area, seems to depend more on the regional competence of the anterior spinal artery than on the number of collateral sources to the cord. Spinal cord injury after cross clamping without adjunctive vascular support has been reported to range from 15% to 25%, depending on the series of cases reviewed.95,96 Proximal-to-distal aortic shunting may alleviate the undesirable hypertension in the aortic distribution proximal to the first clamp and the hypotension in the segments distal to the second clamp. The work of Molina and colleagues95 on dogs indicated, however, that the shunt capacity should provide more than 60% of the baseline descending aortic flow and have a diameter greater than one half of the descending aorta to be effective.
Of particular significance was the study by Svensson and colleagues97 on the blood flow in the baboon spinal cord and its implications in aortic cross clamping. This animal was chosen because its spinal vascularity is similar to humans in that its anterior spinal artery is a continuous vessel without the occasional interruptions noted in some quadrupeds. This study indicated that in baboons, as in humans, the caliber of the anterior spinal artery is often critically narrowed where the thoracic anterior spinal artery joins the lumbar segment of this vessel at their common junction with the arteria medullaris magna. The functional implication is that the shunting of the cross-clamped aorta may help maintain an adequate flow in the lumbosacral sections of the cord but is of little help to the supply of the lower sections of the thoracic cord, owing to the marked discrepancy that usually exists between the anterior spinal artery diameters above and below the junction of the arteria medullaris magna.
In accordance with the hemodynamic principles of Poiseuille’s equation, the resistance to blood flow upward from the arteria medullaris magna junction was more than 50 times greater than the flow resistance downward into the lumbosacral anterior spinal artery in the baboon. Because a series of direct measurements showed that this discrepancy in the anterior spinal artery diameters was even greater in humans, Svensson and colleagues97 concluded that even the lowest segments of the thoracic cord were dependent on a blood flow from the superior end of the thoracic anterior spinal artery despite the shunting.
Intrinsic Venous Drainage of the Spinal Cord
The internal substance of the dorsal half of the cord drains by a centrifugal arrangement of intrinsic vessels that are tributaries, by way of a venous vasa corona, to a large median dorsal longitudinal spinal vein; the ventral half sends tributaries to sulcal veins that empty into a large median ventral longitudinal vein that runs parallel to the anterior spinal artery. Both of these longitudinal vessels are circumferentially connected by a prominent venous vasa corona. This entire system drains into the epidural venous plexus by medullary (previously called radicular) veins that are as infrequent in their distribution as the medullary arteries.98 The proximal sections of the spinal nerve roots drain centripetally into the vasa corona and longitudinal veins of the cord and then to the epidural system via the medullary veins.
Vascularization of the Spinal Nerve Roots
The very long roots of the lumbosacral spinal nerves seemed to be particularly vulnerable because their vascularity was initially believed to be supplied only from their distal ends without the access to the frequent collateral support that is characteristic of peripheral nerves. Because the nerve root fasciculi do not have a strong connective tissue support, it also seemed that the fine vascularity they possessed would be at risk from the repeated tension and relaxation resulting from the flexion and extension of the spine. Parke and colleagues99 and Parke and Watanabe100 showed by vascular injection that the roots receive their arterial supply from both ends (Fig. 2–41; see Fig. 2–36), however, a fact physiologically confirmed by Yamamoto.101
The existence of many redundant coils along the branches of the true radicular arteries ameliorates the stresses that would result from the interfascicular movements that accompany the repeated stretch and relaxation. A significant finding was the occurrence of numerous, relatively large arteriovenous anastomoses throughout the length of the root (Fig. 2–42). These vascular cross connections apparently allow blood flow to be maintained in sections of the root above and below a point of compression. Of particular significance to root nutrition is the work of Rydevik and colleagues100 who, using isotopically labeled methylglucose, showed that approximately 50% of the root nutrition is derived from the ambient cerebrospinal fluid; this necessitates a gauzelike architecture of the radicular pia-arachnoid sheath (Fig. 2–43; see Fig. 2–42B).
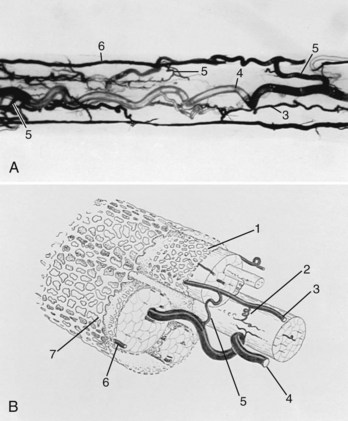
FIGURE 2–42 A, Low-power (×20) transillumination photomicrograph of midsection from part of L4 nerve root that had been treated with hydrogen peroxide after vascular injection with latex–India ink but before clearing in a solution of tributyl-tricresyl phosphates. The peroxidases within the residual blood elements inflated the radicular veins (4) to provide a temporary contrast medium. Note the frequency of the large arteriovenous anastomoses (5) that permitted the latex–India ink to enter the veins. B, Graphic compilation showing structure of a typical lumbosacral nerve root derived from data obtained by injection studies and scanning electron microscopy (see Fig. 2–38). The gauzelike pia-arachnoid membranes permit the cerebrospinal fluid to percolate into nerve tissues and assist metabolic support. Numbers in A and B are common to equivalent structures.
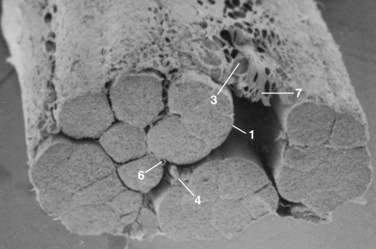
FIGURE 2–43 Scanning electron photomicrograph of section of proximal part of L5 ventral nerve root. The gauzelike pia-arachnoid sheath is very evident. The numbers correspond to the structures labeled in Figure 2–42.
A study by Watanabe and Parke102,103 of chronically compressed roots indicated that the compressed segment is most likely metabolically deprived. It has been suggested that radicular pain is related to root ischemia because a reduction of oxygen intake in patients with neurogenic claudication exacerbates the symptoms.104 The arterial side of the vasa radiculorum seems to be well compensated, however, and maintains a continuity despite severe chronic compression. Further study has indicated that the venous side of the radiculomedullary circulation is more vulnerable.103 Because the roots are part of the central nervous system, the relationships of the arteries to the veins resemble those of the brain more than those of peripheral nerves. The radicular veins do not follow the arterial pattern. They are fewer in number and run a separate and usually deeper (more central) course. Being thin-walled, they are more liable to the spatial restrictions imposed by degenerative changes in the dimensions of the spinal canal and intervertebral foramina and show complete interruption in the chronically compressed root. The metabolically deprived, or inflamed, nerve root becomes hypersensitive to any mechanical deformation, and any additional insult to such a nerve may initiate ectopic impulses that produce pain.
Impedance of the radiculomedullary venous return can occur without topographically related venous constriction. The exacerbation of neurogenic pain in cases in which spinal stenosis has been associated with venous hypertension has been recorded by clinical investigators. LaBan105 and LaBan and Wesolowski106 noted that patients with diminished right-sided heart compliance and spinal stenosis may eventually exhibit neurogenic pain even in static or recumbent situations. They attributed this phenomenon to an increased external pressure on the already sensitized roots by the engorgement of the epidural venous sinuses (see Fig. 2–35), but the venous hypertension alone may be sufficient to impede the venous return from an already compromised radicular circulation. Madsen and Heros107 showed that “arterialization” of spinal veins by abnormal arteriovenous shunts in the region of the conus medullaris exacerbates the neurogenic pain in patients with spinal stenosis. Their hypothesis suggested that a variable combination of increased mechanical constriction by dilated epidural veins and the direct increased resistance to the radicular circulation by the venous hypertension could contribute to the elicitation of pain. Aboulker and colleagues108 also concluded that epidural venous hypertension alone may produce radicular symptoms or cord symptoms or both without adjunctive stenotic compression.
If the intrinsic circulation of the nerve root is impeded in either its arterial input or its venous outflow, the net effect seems to be the same: a neuroischemia of the compressed root segment that may enhance the generation of ectopic nerve impulses. A phenomenon that could be related to radicular venous stasis is the swelling of the disc-distorted nerve root that Takata and colleagues109 showed in CT myelograms. This phenomenon is difficult to explain because extravasated fluids in the root tissues should have free access to the surrounding cerebrospinal fluid. Nevertheless, the fluid balance of the root tissues seems to be altered, particularly in the segment proximal to the level of the offending disc. The intricacies of the hemodynamic relationships responsible for this change remain unknown.
Functional Anatomy of the Spine
The biomechanics of the spine is a very complex and extensive subject. A comprehensive discussion is beyond the scope of this chapter, so the reader is directed to the work of White and Panjabi,110 which is generally regarded as the major book in this field. Because an appreciation of the essential functional relationships of the spinal components does enhance an understanding of their anatomy, however, a brief overview follows.
Biomechanics of the Intervertebral Disc
Were the nucleus pulposus simply a cavity filled with water, it would momentarily act in the same capacity, but the ability to maintain the appropriate quantity of fluid during the continual compression and recovery cycle would be lacking. This ability to absorb and retain relatively large amounts of water is the unique property of the living tissue of the nucleus.111 The essential compound involved in this process is a protein-polysaccharide gel, which through a high imbibition pressure binds nearly nine times its volume of water. It is apparent that the hydrophilia is not a form of biochemical bonding because a quantity of water can be expressed from the nucleus by prolonged mechanical pressure. This accounts for the diurnal decrease in the total length of the spine and its recovery in the supine position at night.
The anulus must receive the ultimate effects of most forces transmitted from one vertebral body to another. Because the major loading of the intervertebral disc is in the form of vertical compression, it may seem paradoxical that the anulus is best constructed to resist tension, but the nucleus transforms the vertical thrust into a radial pressure that is resisted by the tensile properties of the lamellae. Although the basic plan of alternating bands of fibers is one of the obvious sources of the tensile strength of the anulus, this arrangement is not uniform with respect to the directions of the fibers or the degrees of resistance and resilience encountered throughout the anulus. The fibers generally become longer, and the angle of their spiral course becomes more horizontal near the circumference of the disc because it is here that the shearing stresses of vertebral torsions would be most effective. Experimental analysis has also shown that various parts of the anulus do not respond equally to the same degree of tension, and the discrepancies were related to the plane of section and the location of the sample.112 The anulus proved to have the greatest resistance and the greatest recovery in horizontal sections of the peripheral lamellae, whereas vertical and more medial sections were more distensible.
Because the spine acts as a flexible boom to the guidewire actions of the erector spinae muscles, it is essentially the fulcrum of a lever system of the first class, in which the loading has a considerable mechanical advantage. Pure vector analysis has indicated that a theoretical pressure of approximately three fourths of a ton could be applied to a disc when 100 lb is lifted by the hands,14 but this is considerably in excess of the actual pressures achieved. Increased intrathoracic and intra-abdominal pressures alleviate much of the fulcrum compression of the discs by effectively counteracting the load of the anterior lever arm.
The actual pressure variations occurring with postural changes have been recorded by inserting transducers into the third lumbar disc.113,114 This procedure indicated that the internal disc pressure increases from approximately 100 kg in a standing position with the spine erect to 150 kg when the trunk is bent forward and to 220 kg when a 70-kg man lifts a 50-kg weight. It was particularly revealing that the pressure showed a considerable increase when the equivalent maneuvers were repeated in a sitting position, and the weight lifting ultimately created a pressure of 300 kg on the third lumbar disc.
The disc is also “preloaded.” The inherent tensions of the intervertebral ligaments and the anulus exert a pressure of about 15 kg because this weight is required to restore the original thickness of the disc after the ligaments have been divided.100 From a comparative standpoint, this preloading probably offers increased stability to the spine as a functional flexible rod. One is almost induced unconsciously to use teleologic thinking in terms of the vertical thrust resistance when regarding the structure of the disc. In perspective, however, the intervertebral disc shows a consistent morphology in all mammals, yet humans are the only species that truly stand erect. Although analysis of muscular action would most likely show that all mammalian discs must dissipate and transfer axial thrusts, the preloading would enhance the “beam strength” that is obviously necessary in the vertebral column of quadrupeds.
Acknowledgments
1 Bogduk N, Tynan W, Wilson AS. The nerve supply to the human lumbar intervertebral disc. J Anat. 1981;132:39-56.
2 Ebraheim NA, Xu R, Knight T, et al. Morphometric evaluation of lower cervical pedicle and its projection. Spine. 1997;22:1-6.
3 Jasani V, Jaffray D. The anatomy of the iliolumbar vein: A cadaver study. J Bone Joint Surg Br. 2002;84:1046-1049.
4 Parke WW, Watanabe R. The intrinsic vasculature of the lumbosacral spinal nerve roots. Spine. 1985;10:508-515.
5 Scoles PV, Linton AE, Latimer B, et al. Vertebral body and posterior element morphology: The normal spine in middle life. Spine. 1988;13:1082-1086.
1 Francis WR, Fielding JW. Traumatic spondylolisthesis of the axis. Orthop Clin North Am. 1978;9:1011-1027.
2 McCulloch JA, Transfelt EE. Macnab’s Backache. Baltimore: Williams & Wilkins; 1997.
3 Weiner BK, Walker M, Wiley W, et al. The lateral buttress: An anatomic feature of the lumbar pars interarticularis. Spine. 2002;27:E385-E387.
4 An HS, Wise JJ, Xu R. Anatomy of the cervicothoracic junction: A study of cadaveric dissection, cryomicrotomy and magnetic resonance imaging. J Spinal Disord. 1999;12:519-525.
5 Ebraheim NA, Xu R, Knight T, et al. Morphometric evaluation of lower cervical pedicle and its projection. Spine. 1997;22:1-6.
6 Pait TG, McAllister PV, Kaufman HH. Quadrant anatomy of the articular pillars (lateral cervical mass) of the cervical spine. J Neurosurg. 1995;82:1011-1014.
7 Xu R, Burgar A, Ebraheim NA, et al. The quantitative anatomy of the laminas of the spine. Spine. 1999;24:107-113.
8 Chaynes P, Sol JC, Vaysse P, et al. Vertebral pedicle anatomy in relation to pedicle screw fixation: A cadaver study. Spine. 2001;23:85-90.
9 Cinotti G, Gumina S, Ripani M, et al. Pedicle instrumentation in the thoracic spine: A morphometric and cadaveric study for placement of screws. Spine. 1999;24:114-119.
10 Scoles PV, Linton AE, Latimer B, et al. Vertebral body and posterior element morphology: The normal spine in middle life. Spine. 1988;13:1082-1086.
11 Olszewski AD, Yaszemski MJ, White AA. The anatomy of the human lumbar ligamentum flavum: New observations and their surgical implications. Spine. 1996;21:2307-2312.
12 von Luschka H. Die Halbgelenke des menschlichen Korpers. Berlin: Karpess; 1858.
13 Beadle OA. The Intervertebral Discs Special Report No. 160. London: Medical Research Council. 1931:pp 6-9.
14 Bradford DL, Spurling RG. The Intervertebral Disc. Springfield, IL: Charles C Thomas; 1945.
15 Humzah MD, Soames RW. Human intervertebral disc: Structure and function. Anat Rec. 1988;229:337-356.
16 Bick EM. The osteohistology of the normal human vertebra. J Mt Sinai Hosp. 1952;19:490-527.
17 Aeby C. Die Alterverschiedenheiten der menschlichen Wirbelsaule. Arch Anat Physiol (Anat Abst). 1879;10:77.
18 Dommissee G. Morphological aspects of the lumbar spine and lumbosacral regions. Orthop Clin North Am. 1975;6:163-175.
19 Wiltse LL. Anatomy of the extradural compartments of the lumbar spinal canal: Peridural membrane and circumneural sheath. Radiol Clin North Am. 2000;38:1177-1206.
20 Ugur HC, Attar A, Uz A, et al. Surgical anatomic evaluation of the cervical pedicle and adjacent neural structures. Neurosurgery. 2000;47:1162-1168.
21 Ugur HC, Attar A, Uz A, et al. Thoracic pedicle: Surgical anatomic evaluation and relations. J Spinal Disord. 2001;14:39-45.
22 Ebraheim NA, Xu R, Darwich M, et al. Anatomic relations between the lumbar pedicle and the adjacent neural structures. Spine. 1997;15:2338-2341.
23 Hogan Q, Toth J. Anatomy of the soft tissues of the spinal canal. Reg Anesth Pain Med. 1999;24:303-310.
24 Kobrine AI, Doyle DF, Rizzoli HV. Spinal cord blood flow as affected by changes in systemic arterial blood pressure. J Neurosurg. 1976;44:12-15.
25 Golub GS, Silverman B. Transforaminal ligaments of the lumbar spine. J Bone Joint Surg Am. 1969;51:947-956.
26 Park HK, Rudrappa S, Dujovny M, et al. Intervertebral foraminal ligaments of the lumbar spine: Anatomy and biomechanics. Child Nerv Syst. 2001;4-5:275-282.
27 Grimes PF, Massie JB, Garfin SR. Anatomic and biomechanical analysis of the lower lumbar foraminal ligaments. Spine. 2000;25:2009-2014.
28 Alleyne CH, Cawley CM, Barrow DL, et al. Microsurgical anatomy of the dorsal cervical nerve roots and the cervical dorsal root ganglion/ventral root complexes. Surg Neurol. 1998;50:213-218.
29 Kadish LJ, Simmons EH. Anomalies of the lumbosacral nerve roots. J Bone Joint Surg Br. 1984;66:411-416.
30 Kikuchi S, Hasue M. Anatomic features of the furcal nerve and its clinical significance. Spine. 1986;11:1002-1007.
31 Piacsecka-Kacperska A, Gladykowska-Rzeczycka J. The sacral plexus in primates. Folia Morphol (Warsz). 1972;31:21-31.
32 Parke WW, Watanabe R. Lumbosacral intersegmental epispinal axons and ectopic ventral nerve rootlets. J Neurosurg. 1987;67:269-277.
33 Hasue M, Kunogi J, Konno S, et al. Classification by position of dorsal root ganglia in the lumbosacral region. Spine. 1989;14:1261-1264.
34 Kikuchi S, Hasue M. Combined contrast studies in lumbar spine diseases. Spine. 1988;13:1327-1331.
35 Bogduk N, Tynan W, Wilson AS. The nerve supply to the human lumbar intervertebral disc. J Anat. 1981;132:39-56.
36 Bogduk N, Windsor M, Inglis A. The innervation of the cervical intervertebral discs. Spine. 1988;13:2-8.
37 Hirsch C. Studies on mechanism of low back pain. Acta Orthop Scand. 1953;22:184-231.
38 Jung A, Brunschwig A. Recherches histologiques sur l’innervation des articulations et des corps vertebreaux. Presse Med. 1932;40:316-317.
39 Larmon AW. An anatomic study of the lumbosacral region in relation to low back pain and sciatica. Ann Surg. 1944;119:892.
40 Malinsky J. The ontogenetic development of nerve terminations in the intervertebral discs of man. Acta Anat. 1959;38:96-113.
41 Nade S, Bell S, Wyke BD. The innervation of the lumbar spine joints and its significance. J Bone Joint Surg Br. 1980;62:225-261.
42 Wiberg G. Back pain in relation to nerve supply of intervertebral disc. Acta Orthop Scand. 1949;19:211-221.
43 Parke WW. Applied anatomy of the spine. In: Rothman RH, Simeone FA, editors. The Spine. Philadelphia: WB Saunders; 1982:18-51.
44 Groen GJ, Baljet B, Drukker J. The nerves and nerve plexuses of the human vertebral column. Am J Anat. 1990;188:282-296.
45 Pedersen HE, Blunck CFJ, Gardner E. The anatomy of the lumbosacral posterior rami and meningeal branches of spinal nerves (sinuvertebral nerves). J Bone Joint Surg Am. 1956;38:377-391.
46 Hirsch C, Inglemark B, Miller M. The anatomical basis for low back pain. Acta Orthop Scand. 1963;33:1-17.
47 McCouch GP, During ID, Ling TH. Location of receptors for tonic reflexes. J Neurophysiol. 1951;14:191-195.
48 Stilwell DL. The nerve supply of the vertebral column and its associated structures in the monkey. Anat Rec. 1956;125:139-169.
49 Yoshizawa H, O’Brien JP, Thomas-Smith W, et al. The neuropathology of intervertebral discs removed for low back pain. J Pathol. 1980;132:95-104.
50 Kimmel DL. Innervation of the spinal dura and the dura of the posterior cranial fossa. Neurology. 1986;11:800-809.
51 Cyriax J. Dural pain. Lancet. 1978;1:919-921.
52 Groen GJ, Baljet B, Drukker J. The innervation of the spinal dura mater: Anatomy and clinical implications. Acta Neurochir. 1988;92:39-46.
53 Parke WW, Watanabe R. Adhesions of the ventral lumbar dura: An adjunct source of discogenic pain? Spine. 1990;15:300-303.
54 Blikra G. Intradural herniated lumbar disc. J Neurosurg. 1969;31:676-679.
55 Junghanns H. Der Lumboscralwinkel. Dtsch Z Chit. 1929;213:332.
56 Schmorl G, Junghanns H. The Human Spine in Health and Disease. New York: Grune & Stratton; 1959.
57 DePalma AF, Rothman RH. The Intervertebral Disc. Philadelphia: WB Saunders; 1970.
58 Brown MD. The Pathophysiology of the Intervertebral Disc: Anatomical, Physiological and Biomedical Considerations. Philadelphia: Jefferson Medical College; 1969.
59 Maroudas A. Nutrition and metabolism of the intervertebral disc. In: Ghosh P, editor. The Biology of the Intervertebral Disc. Boca Raton, FL: CRC Press, 1988.
60 Holm S, Maroudas A, Urban JPG, et al. Nutrition of the intervertebral disc: An in vivo study of solute transport. Clin Orthop. 1977;129:104-114.
61 Holm S, Maroudas A, Urban JPG, et al. Nutrition of the intervertebral disc: Solute transport and metabolism. Connect Tissue Res. 1981;8:101-110.
62 Maroudas A, Nachemson A, Stockwell RA, et al. Factors involved in the nutrition of human lumbar intervertebral disc: Cellularity and diffusion of glucose in vitro. J Anat. 1975;120:113-130.
63 Ferguson WP. Some observations on the circulation in fetal and infant spines. J Bone Joint Surg. 1950;32:640-645.
64 Willis TA. Nutrient arteries of the vertebral bodies. J Bone Joint Surg. 1949;31:538-541.
65 Crock HV, Yoshizawa H. The Blood Supply of the Vertebral Column and Spinal Cord in Man. New York: Springer-Verlag; 1977.
66 Milen MT, Bloom DA, Culligan J, et al. Albert Adamkiewicz (1850-1921)—his artery and its significance for the retroperitoneal surgeon. World J Urol. 1999;17:168-170.
67 Parke WW. The vascular relations of the upper cervical vertebrae. Orthop Clin North Am. 1978;9:879-889.
68 Schiff DCM, Parke WW. The arterial supply of the odontoid process. Anat Rec. 1972;172:399-400.
69 Jasani V, Jaffray D. The anatomy of the iliolumbar vein: a cadaver study. J Bone Joint Surg Br. 2002;84:1046-1049.
70 Kaisary AV, Smith P. Spinal cord ischemia after ligation of both internal iliac arteries during radical cystoprostectomy. Urology. 1985;25:395-397.
71 Breschet G. Essai sur les Veines der Rachis. Paris: Mequigon-Morvith; 1819.
72 Batson OV. The function of the vertebral veins and their role in the spread of metastases. Am Surg. 1940;112:138-145.
73 Clemens HJ. Die Venesysteme der menschlichen Wirbelsaule. Berlin: Walter de Gruyter; 1961.
74 Chaynes P, Verdie JC, Moscovici J, et al. Microsurgical anatomy of the internal vertebral venous plexuses. Surg Radiol Anat. 1998;20:47-51.
75 Parke WW, Valsamis MP. The ampulloglomerular organ: An unusual neurovascular complex in the suboccipital region. Anat Rec. 1967;159:193-198.
76 Parke WW, Rizzoli HV, Brown MD. The pharyngovertebral veins: An anatomic rationale for Grisel’s syndrome. J Bone Joint Surg Am. 1984;66:568-574.
77 Wetzel FT, LaRocca H. Grisel’s syndrome: A review. Clin Orthop. 1989;240:141-152.
78 Corbib JL. Anatomie et Pathologie Arterielles de la Moelle. Paris: Masson et Cie; 1961:pp 787-796.
79 Brewer LA, Fosburg RG, Mulder GA, et al. Spinal cord complications following surgery for coarctation of the aorta. J Thorac Cardiovasc Surg. 1972;64:368-379.
80 Lazorthes G, Gouaze A, Zadeh JO, et al. Arterial vascularization of the spinal cord. J Neurosurg. 1971;35:253-262.
81 Marcus ML, Heistad DD, Ehrardt JC, et al. Regulation of total and regional spinal cord blood flow. Circ Res. 1977;41:128-134.
82 Parke WW, Whalen JL, Bunger PC, et al. Intimal musculature of the lower anterior spinal artery. Spine. 1995;20:2073-2079.
83 Parke WW. Correlative anatomy of cervical spondylotic myelopathy. Spine. 1988;13:831-837.
84 Dommissee GF. The Arteries and Veins of the Human Spinal Cord from Birth. Edinburgh: Churchill-Livingstone; 1975.
85 Lasjaunias P, Vallee B, Person H, et al. The lateral artery of the upper cervical spinal cord. J Neurosurg. 1985;63:235-241.
86 Gillilan LA. The arterial blood supply of the human spinal cord. J Comp Neurol. 1958;110:75-103.
87 Hassler O. Blood supply to human spinal cord. Arch Neurol. 1966;15:302-307.
88 Herren RY, Alexander L. Sulcal and intrinsic blood vessels of human spinal cord. Arch Neurol Psychiatry. 1939;41:678-683.
89 Kadyi H. Über die Blutgefasse des menschlichen Ruckenmarkes: Nach einer im XV Bande der Denkschriften d. math-naturw. Cl. d. Akad. d. Wissensch. Krakau erschienen Morphology, aus dem Polnischen Ubersaatz vom Verfasser. Lemberg: Grubrnowicz & Schmidt; 1889.
90 Turnbull IM, Brieg A, Hassler O. Blood supply of cervical spinal cord in man. J Neurosurg. 1966;24:951-965.
91 Ireland WP, Fletcher TF, Bingham C. Quantification of microvasculature in the canine spinal cord. Anat Rec. 1981;200:103-113.
92 Feeney JF, Watterson RL. The development of the vascular pattern within the walls of the central nervous system of the chick embryo. J Morphol. 1946;78:231-303.
93 Lobosky JM, Hitchon PW, Torner JC, et al. Spinal cord autoregulation in the sheep. Curr Surg. 1984;41:264-267.
94 Parke WW. Arteriovenous anastomoses in the spinal cord: Probable role in blood flow autoregulation [abstract]. Anat Rec. 1989;223:87A.
95 Molina JE, Cogordon J, Einzig S, et al. Adequacy of ascending-descending aorta shunt during cross-clamping of the thoracic aorta for prevention of spinal cord injury. J Thorac Cardiovasc Surg. 1985;90:126-136.
96 Wadouh F, Arndt CF, Opperman E, et al. The mechanism of spinal cord injury after simple and double aortic cross-clamping. J Thorac Cardiovasc Surg. 1986;92:121-127.
97 Svensson LG, Rickards E, Coull A, et al. Relationship of spinal cord blood flow to vascular anatomy during thoracic aorta cross-clamping and shunting. J Thorac Cardiovasc Surg. 1986;91:71-78.
98 Gillilan LA. Veins of the spinal cord. Neurology. 1970;20:860-868.
99 Parke WW, Gammel K, Rothman RH. Arterial vascularization of the cauda equina. J Bone Joint Surg Am. 1981;63:53-62.
100 Parke WW, Watanabe R. The intrinsic vasculature of the lumbosacral spinal nerve roots. Spine. 1985;10:508-515.
101 Yamamoto H. Quantitative measurements of blood flow in cauda equina in spinal cords of monkeys by using radioactive microspheres. J Jpn Coll Angiol. 1982;22:35-42.
102 Watanabe R, Parke WW. The vascular and neural pathology of lumbosacral spinal stenosis. J Neurosurg. 1986;65:64-70.
103 Watanabe R, Parke WW. Structure of lumbosacral spinal nerve roots: Anatomy and pathology in spinal stenosis. J Clin Orthop Surg (Jpn). 1987;22:529-539.
104 Evans JG. Neurogenic intermittent claudication. BMJ. 1964;2:985-987.
105 LaBan MM. “Vesper’s curse”: Night pain, the bane of Hypnos. Arch Phys Med Rehabil. 1984;65:501-504.
106 LaBan MM, Wesolowski DP. Night pain associated with diminished cardiopulmonary compliance. Am J Phys Med Rehabil. 1988;67:155-160.
107 Madsen JR, Heros RC. Spinal arteriovenous malformations and neurogenic claudication. J Neurosurg. 1988;68:793-797.
108 Aboulker J, Bar D, Marsault C, et al. L’hypertension veineuse intra-rachidienne par anomalies multiples du système cave: Une cause majeure de souffrance médullaire. Clin Obstet Gynecol. 1977;103:1003-1015.
109 Takata K, Inoue S, Takashi K, et al. Swelling of the cauda equina in patients who have herniation of a lumbar disc. J Bone Joint Surg Am. 1988;70:361-368.
110 White A, Panjabi M. Clinical Biomechanics of the Spine, 2nd ed. Philadelphia: JB Lippincott; 1990.
111 Puschel J. Der Wassergehalt normaler and degenerierter Zwischenwirbelscheiben. Beitr Pathol Anat. 1930;84:123-130.
112 Galante JO. Tensile properties of the human lumbar annulus fibrosus. Acta Orthop Scand. 1967;100(Suppl):1-91.
113 Nachemson A. The load on lumbar discs in different positions of the body. Clin Orthop. 1966;45:107-122.
114 Petter CK. Methods of measuring the pressure of intervertebral discs. J Bone Joint Surg. 1933;15:365.