CHAPTER 41 ANATOMY AND PHYSIOLOGY OF CEREBRAL AND SPINAL CORD CIRCULATION
Of all the mammalian organs, the central nervous system is the most privileged and protected. Claude Bernard developed the now near-axiomatic concept of the maintenance of the “milieu interieur.” It is essential to recognize that the stability of the internal environment of the brain is primordial and that the homeostasis of other organs is subordinated to the vital stability of the neuronal environment in the central nervous system. Before entering into the anatomical and the physiological details that are specific to the brain, it is worth reflecting on the major general systems that protect the body—above all, the central nervous system.
ANATOMY OF THE CEREBRAL CIRCULATION
Arterial Supply
The arterial supply to the human brain consists of four major afferent arterial trunks: two internal carotid and two vertebral arteries. The internal together with the external carotid arteries derive from the common carotid artery. In humans, the carotid arteries are quantitatively more important; each contributes approximately 40% to the total perfusion of the brain. The internal carotid arteries enter the cranial cavity through the os petrosum. The vertebral arteries enter the cranial cavity through the foramen magnum, where, after traversing the anterolateral aspect of the medulla oblongata, they fuse to form the basilar artery at the level of the pontomedullary junction (Fig. 41-1). This artery unites with the two internal carotids to form, at the base of the brain, an equalizing distributor named the circle of Willis (see Fig. 41-1). Having fused with the basilar artery, each internal carotid artery divides into four major branches: the anterior cerebral, the middle cerebral, the anterior choroidal, and the posterior communicating arteries. The latter anastomose with the posterior cerebral arteries, which originate from the basilar artery to complete the circle of Willis (see Fig. 41-1). These major cerebral arteries divide into progressively smaller arteries, which, in turn, enter the brain parenchyma at a right angle to the surface of the brain to supply blood to specific regions. The anterior cerebral arteries irrigate the frontal pole and the medial aspects of both frontal and parietal lobes, the corpus callosum, the anterior limb of the internal capsule, and the most rostral part of the caudate nucleus and the putamen. The middle cerebral arteries supply most of the lateral aspects of the cerebral hemispheres, as well as portions of the caudate nucleus and the putamen. The anterior choroidal arteries supply several structures such as the choroid plexus of the lateral ventricle, the optic tract, the hippocampus, the tail of the caudate nucleus, and the amygdala. The posterior communicating arteries supply the genu of the corpus callosum, part of the posterior limb of the internal capsule, the rostral thalamus, and the wall of the third ventricle. The posterior cerebral arteries supply the inferior and medial aspects of the temporal and the occipital lobes, parts of the hippocampus, and the thalamus (Fig. 41-2). Before giving rise to the posterior cerebral arteries, the basilar artery sends several branches to supply the cerebellum and the brainstem (see Fig. 41-1).
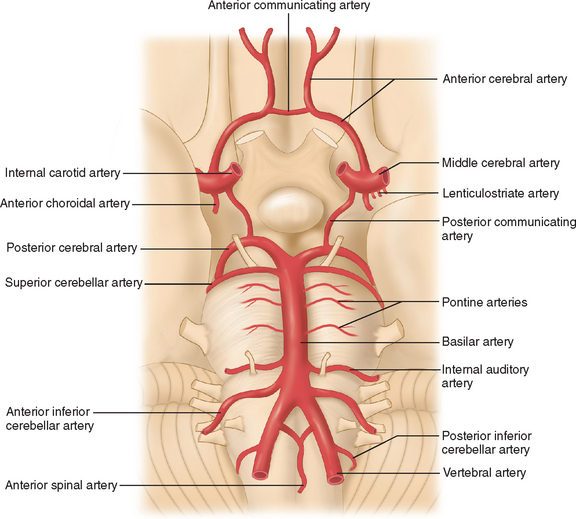
Figure 41-1 Basal view of major cerebral arteries and the circle of Willis.
(From Sokoloff L: Anatomy of the Cerebral Circulation. In Welch KMA, Caplan LR, Reis DJ, et al, eds: Primer on Cerebrovascular Diseases. Academic Press, 1997.)
Collateral Blood Supply of the Brain
Several types of anastomoses are found in the cerebral circulation.1 The following are the most important.
Circle of Willis
As mentioned, the circle of Willis is an anastomosis between the anterior and the posterior circulation via the anterior and the posterior communicating arteries (see Fig. 41-1). Considerable intraspecies and interspecies variability exists in the anatomy of the circle of Willis. In humans, a symmetrical circle is found in only 50% of brains.2 In addition, in most species other than primates, the anterior cerebral communicating artery is absent and the anterior cerebral arteries fuse earlier to form the pericallosal artery.3 This anastomotic ring protects from the disastrous consequences of occlusion of a single supply vessel to the brain. However, under physiological conditions, the blood from the internal carotid and the basilar arteries does not mix because the blood pressure in each arterial trunk is almost identical.
External Carotid-Internal Carotid Anastomoses
In humans, this anastomotic pathway is relatively negligible. The ophthalmic artery is the most significant conduit that is capable of bridging the external and internal circulations. However, in many species such as the dog, cat, and sheep, rich and complex connections exist between the internal and external carotid arteries via a structure termed the rete mirabile.3
Leptomeningeal Anastomoses
The leptomeningeal anastomoses, organized in the subarachnoid space between the arterial boundary zones, represent connections between distal branches of major cerebral arteries (i.e., between the anterior cerebral and middle cerebral arteries and between the middle cerebral and the posterior cerebral arteries) (Fig. 41-3). These arterial boundary zones, also termed “watershed zones,” are especially susceptible to damage following any generalized decrease in blood flow as in the case of severe systemic hypotension.
Venous Drainage
The venous circulation of the central nervous system is particular in that (1) the veins do not run parallel to arteries as in many other organs and (2) the major fraction of blood that drains the brain is collected in the dural sinuses, which represent the final intracranial collecting blood vessels.4 Briefly, there are three groups of valveless vessels that allow for drainage. These are the superficial cortical veins located on the surface of the cortex, the deep or central veins, and the venous sinuses within the dura (Fig. 41-4). The superficial veins convey blood from the cortex and the adjacent white matter and empty into the dural venous sinuses. The deep cerebral veins drain blood in a centripetal direction from the deep white matter, the basal ganglia, and the diencephalon toward the lateral ventricles. Large subependymal veins empty into the internal cerebral and basal veins, which unite and contribute to the formation of the great cerebral vein also known as the “vein of Galen.” The cerebral venous system is also exceptional in that these vessels are endowed with arachnoid villae, which allow cerebrospinal fluid and various metabolites to drain into the systemic circulation.
Blood Supply to the Spinal Cord
A network of irregular anastomosing arteries connects the ventral spinal and dorsolateral arteries. The dorsolateral arteries and their anastomosing branches give rise to radial arteries that supply both gray and white matter in the spinal cord. Each body segment has paired spinal arteries that give rise to a dorsal root artery and a ventral root artery, which follow the nerve connections on each side of the cord. These arteries feed into a spinal arterial ring that surrounds the cord at the level of each intervertebral foramen. The venous architecture of the spinal cord is considerably more variable than the corresponding arterial distribution, and the blood vessels are more tortuous. There tends to be a prominent midline vein that in general follows the course of the posterior median sulcus. Otherwise, the veins follow the same pattern as the intrinsic, radial, and segmental arteries (for details, see Shamji et al5).
Microcirculation
The main arteries enter the subarachnoid space and divide many times before penetrating the substance of the brain to form smaller arterioles and capillaries. The smallest pial arteries enter the brain parenchyma at a right angle to the surface of the brain. These pial arteries are formed by endothelial and smooth muscle cell layers as well as an outer layer of cells, termed the adventitia, which contains collagen, fibroblasts, and perivascular nerves. The penetrating arterioles are surrounded by an invagination of the pia matter, creating a perivascular space (Virchow-Robin space) that is contiguous with the subarachnoid space. As the arterioles penetrate deeper into the brain, this space disappears and the vascular basement membrane comes into direct contact with the astrocytic end-feet (intracerebral arterioles and capillaries). Capillaries are formed by one layer of endothelial cells and show a marked heterogeneous distribution that is correlated with synaptic density and local energy metabolism.6,7 In most regions of the brain, endothelial cells are unique in that they lack fenestrations and are interconnected by specific intercellular junctions, known as tight junctions. These morphological features, in conjunction with metabolic activity that is essentially limited to cerebral endothelial cells, constitute the blood-brain barrier that excludes large molecules, neurotransmitters, and toxins by both forming a physical barrier and lacking the typical transport mechanisms that operate in blood vessels most in other regions of the body. It should be emphasized, however, that the endothelial cell is more than the anatomical interface between cerebrovascular muscle and the blood; it plays an important functional role in the regulation of cerebral blood flow.8 Indeed, the cerebrovascular endothelium is able to produce a variety of vasodilatatory and constrictory agents. Intraparenchymal blood vessels are almost completely ensheathed by astrocyte processes. Recent reports indicate that astrocytes are involved in local regulation of blood supply in response to neuronal activation.9,10 In the central nervous system, pericytes are found and are closely apposed to the abluminal surface of the capillaries of which they cover, on average, 25%. The function of pericytes is not well known, but some studies suggest that they may influence the diameter of capillaries because they display contractile properties.11
One distinguishing feature of the cerebrovascular bed is the presence of a rich and complex innervation.12 Large intracranial and pial vessels are densely innervated by perivascular nerves that originate from autonomic and sensory ganglia (extrinsic innervation) and contain many agents that can potentially modify vascular tone. Intracerebral arterioles and capillaries are contacted by neural processes that originate from local interneurons or from central pathways (intrinsic innervation). These processes also contain many vasoactive substances. These neurally produced transmitters and modulators are likely to participate in the control of the microvascular tone and, thereby, local cerebral blood flow.
Physiology of the Cerebral Circulation
Pressure-Flow Relationships
If the cerebral vascular bed was a system of nondistensible pipes, cerebral blood flow (F) would be, according to Ohm’s law, a simple function derived from the perfusion pressure (the difference between arterial inflow and downstream pressure ΔP) divided by the resistance to flow along these pipes (F = ΔP/R). Resistance to flow is determined by the caliber of the vascular segment, its length, and the nature of the fluid that flows along it. By analogy to Poiseuille’s equation that applies to a rigid system of tubing perfused by a newtonian fluid:
where F is flow, r is vascular radius, ΔP is the pressure gradient between inflow and outflow, η is viscosity, and L is length. Although this equation cannot be totally applicable to the cerebrovascular bed, because it is not a rigid system, its effective length is not known, and blood is a non-newtonian fluid, Poiseuille’s law can reasonably describe the fundamental relationships between cerebral blood flow, perfusion pressure, and resistance. Of great interest, this equation emphasizes that cerebral blood flow is related to the fourth power of vessel radius; thus even minor changes in arterial diameter have a significant impact on cerebral blood flow.
Cerebral perfusion pressure is the difference between intra-arterial pressure where the vessels enter the subarachnoid space and pressure in the thin-walled veins in the subarachnoid space. Venous pressure changes in parallel to intracranial pressure and is normally 2 to 5 mm Hg higher than intracranial pressure. Under physiological conditions, the intracranial pressure is determined by the volume of three compartments: brain parenchyma, quantity of cerebrospinal fluid, and intravascular blood pool. Because of the rigidity of the cranium, increases in the size of one component of the intracranial contents must be accompanied by removal of an equivalent amount of another otherwise intracranial pressure will increase.13
Cerebral Autoregulation
Autoregulation of blood flow is a regulatory mechanism that allows blood flow in a vascular bed to remain relatively constant during variations of arterial pressure. This is particularly well developed in the brain and plays an important protective role against the danger of hypoxia at low perfusion pressure and the risk of brain edema at higher arterial pressure. Indeed, in normotensive humans, cerebral blood flow remains relatively but not absolutely constant over a range of perfusion pressures (approximately 60 to 160 mm Hg) (Fig. 41-5). These two values determine the lower and the upper limits of autoregulation, respectively. The relative constancy of cerebral blood flow during autoregulation is due to the variations of cerebrovascular resistance (Fig. 41-6). Once the limits of autoregulation are reached, cerebral blood flow increases or decreases passively with concomitant increases or decreases in perfusion pressure (see Fig. 41-5). Nonetheless, it should be emphasized that the lower limit of autoregulation does not correspond to maximal vasodilatation because cerebral resistance arteries continue to dilate to some degree below that limit.14 When perfusion pressure decreases below the lower limit of autoregulation, cerebral blood flow decreases in parallel. However, the brain oxygen metabolism remains essentially unaffected because of an increase in oxygen extraction fraction from blood. In this stage, qualified as oligemia, no clinical symptoms of functional disruption are observed. However, further decreases in cerebral perfusion pressure further compromise cerebral blood flow, and so when the oxygen extraction fraction reaches the limit of 100% it becomes insufficient to satisfy the metabolic demands of the cerebral tissues. This stage, defined as ischemia, results in functional disturbance and, depending on its duration, neuronal death may occur.15,16
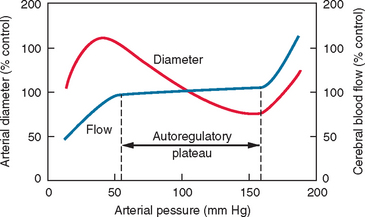
Figure 41-5 Schematic representation of the autoregulation of cerebral blood flow.
(From Chillon JM, Baumbach GL: Autoregulation of cerebral blood flow. In Welch KMA, Caplan LR, Reis DJ, et al, eds: Primer on Cerebrovascular Diseases. New York: Academic Press, 1997.)
When mean arterial pressure increases above the upper limit of autoregulation, resistance arteries in the brain cannot sustain vasoconstriction. An early sign is the appearance of the “sausage string” phenomenon characterized by an alternating pattern of dilated arterial segments with focal regions of constriction. The dilated segments represent regions of passive dilatation, and the constricted segments, regions of sustained autoregulation. Further increases in cerebral perfusion pressure result in general arteriolar dilatation and cerebral blood flow increases passively. This is accompanied by damage to the cerebrovascular endothelium and disruption of the blood-brain barrier, which leads to extravasation of plasma proteins through the vessel wall and subsequent edema.14
The limits of autoregulation are not fixed but vary with physiological stimuli and in disease states. Activation of the sympathetic nerves results in upward shift of both the lower and upper limits of autoregulation—a potentially protective response because acute elevations in arterial pressure are usually accompanied by sympathetic activation. The autoregulatory plateau is shifted to higher values in patients with chronic hypertension. This protective response can have deleterious effects if there is significant hypotension, when symptoms of ischemia may occur at a relatively higher arterial pressure.14
The mechanisms underlying cerebral blood flow autoregulation are not definitively understood. Three classic hypotheses involve neurogenic, myogenic, and metabolic factors. According to the myogenic hypothesis, smooth muscle in the resistance arteries directly contracts or relaxes in response to increases or decreases in perfusion pressure respectively. The rapidity of the autoregulatory response supports a myogenic hypothesis.17 Autoregulation is preserved in animals that have undergone sympathetic and parasympathetic denervation, suggesting that this factor is not of primary importance. Autonomic nerves, however, may play a role by modulating autoregulation. Indeed, it has been shown that sympathetic stimulation or parasympathetic denervation results in a shift of the lower autoregulatory limit toward higher levels of blood pressure.14,18 The metabolic hypothesis states that reductions in cerebral blood flow stimulate the release of vasoactive sub stances from the brain, which in turn stimulates the dilatation of cerebral resistance arteries. Several candidates for this role have been proposed—including carbon dioxide, hydrogen ions, oxygen, adenosine, potassium, and calcium—but no definitive role has been demonstrated for any of these.19
Neurogenic Regulation of the Cerebral Circulation
As mentioned, cerebral vessels are endowed with a dense and complex innervation that originates from the autonomic nerves (sympathetic, parasympathetic, and sensory nerves) as well as from intracerebral neurons. This innervation involves a variety of transmitters of different chemical natures, which have potent vascular actions (Table 41-1). The role and influence of the perivascular nerves on cerebral blood flow regulation have been the subject of considerable controversy. The description of their actions is beyond the scope of this chapter; the reader can refer to published reviews.20 Here, we briefly illustrate the concepts by taking the cholinergic system as an example.
TABLE 41-1 Vasoactive Transmitters Implicated in Neurovascular Regulation
Transmitters | Effect of Stimulation on Cerebral Blood Flow |
---|---|
Norepinephrine | − |
Acetylcholine | + |
Serotonin | +/− |
Dopamine | + |
Nitric oxide | + |
Vasoactive intestinal peptide | + |
Neuropeptide Y | − |
Pituitary adenylate cyclase–activating polypeptide | + |
Substance P | + |
Calcitonin gene–related peptide | + |
Neurokinin A | + |
+, −, and +/− indicate increase, decrease, and both increase and decrease in cerebral blood flow, respectively.
Morphological and functional evidence exists to support a role of cholinergic neurons in the regulation of local cerebral blood flow. Major cerebral arteries and small pial vessels are innervated by parasympathetic cholinergic nerves that originate mainly from the sphenopalatine and the otic ganglia. However, vessels located within the cerebral cortex mainly receive projections from cholinergic neurons located in the basal forebrain.21 Electrical or chemical stimulation of these intrinsic cholinergic neurons has been shown to induce a marked vasodilatation and subsequently a cerebral blood flow increase in the cerebral cortex. These vascular effects are reduced by the administration of atropine, an antagonist of muscarinic receptors, and potentiated by the administration of physostigmine, an inhibitor of acetylcholine esterase—findings that support the involvement of acetylcholine in neurovascular regulation.22 Moreover, Vaucher and colleagues23 demonstrated that the vasodilatation induced by stimulation of cholinergic neurons of the basal forebrain was not accompanied by any change in brain metabolism. Overall, these studies indicate that the changes in perfusion cannot be attributed to increased metabolic activity but rather to a direct action of cholinergic neurons on cerebral blood vessels. Another argument to support the involvement of the cholinergic system in the regulation of intraparenchymal vessels is that lesions of the basal forebrain result in considerable reduction of cerebral blood flow in the cerebral cortex.24 Nonetheless, the significance of the cholinergic system in the regulation of local blood flow in response to cerebral activation is not fully investigated.22
Apart from the direct innervation of cerebral vessels, a neurogenic control of blood flow can also be produced by stimulation of neurotransmitter receptors. As an example, activation of glutamate receptors induces vasodilatation and increase of cerebral blood flow. These effects are mediated by vasoactive factors whose synthesis is triggered by the changes in intracellular Ca2+ associated with the activation of glutamatergic receptors. The increase in Ca2+ activates Ca2+-dependent enzymes that produce potent vasodilators such as nitric oxide and certain arachidonic acid products (see Fig. 41-6).25
A glutamate-induced Ca2+ increase activates the neuronal isoform of nitric oxide synthase (nNOS), which produces nitric oxide. The vasodilatation produced by the topical application of N-methyl-D-aspartate or glutamate is reduced by nNOS inhibitors. Moreover, the cortical cerebral blood flow increase induced by sensory stimulation is generally associated with nitric oxide release and is attenuated by nNOS inhibitors.25 These findings indicate an important role for nitric oxide in the mechanisms of vasodilatation seen during functional activation.
An increase in intracellular Ca2+ evoked by glutamate also activates phospholipase A2, leading to production of arachidonic acid. Arachidonic acid is then metabolized by the cyclooxygenase (COX) pathway, producing vasodilatatory prostaglandins. Functionally, the cerebral blood flow increase evoked by somatosensory stimulation is attenuated by COX-2 inhibitors or in COX-2–null mice, whereas COX-1 does not participate in this response.26 The COX-2 metabolites responsible for vasodilatation are likely to involve vasodilatatory prostaglandins. Other arachidonic acid products involved in functional hyperemia include metabolites of the P450 pathway, such as epoxyeicosatrienoic acids.10,27 There is a growing body of evidence to show that neurovascular regulation of cerebral vessels implicates not only neurons but also glial cells.10,25 Zonta and associates28 showed, through the use of brain slices, that neuronal activation induces glutamate release, which activates metabotropic glutamate receptors located on cortical astrocytes, leading to increases in Ca2+ concentrations in perivascular end-feet, which in turn cause arteriolar dilatation by release of vasoactive cyclooxygenase products (see Fig. 41-6). Similar results were obtained in studies performed in mice using in vivo imaging, further supporting the pivotal role of astrocytes in cerebral blood flow regulation in response to neuronal synaptic activity.29
Metabolic Regulation of the Cerebral Circulation
According to the metabolic hypothesis of regulation of blood flow, neurons release products of neuronal activity that diffuse to the vasculature and result in vasodilatation. This concept of coupling was introduced in a seminal paper by Roy and Sherrington in 1890,30 in which they proposed that “the chemical products of cerebral metabolism … can cause variations of the caliber of the cerebral vessels.” Several factors have been implicated in this type of regulation, including vasoactive ions (K+ and H+), CO2, lactate, and adenosine (see Fig. 41-6). It could well be that the co-release of two or several factors is necessary to meaningfully effect changes in cerebrovascular tone.
Potassium
Potassium ions are released by the extracellular ionic currents induced by action potentials and synaptic transmission. It is well known that increases in extracellular K+ up to 10 mM cause dilatation of arterioles both in vitro and in vivo.31,32 This effect is mediated by the opening of K+ channels on the membrane of arterial smooth muscle cells, leading to their hyperpolarization and subsequent relaxation.8
Carbon Dioxide
The effect of carbon dioxide on the cerebral vasculature is one of the most pronounced and reproduced phenomena observed in the cerebral circulation. Hypercapnia causes cerebral vasodilatation with a cerebral blood flow increase and hypocapnia causes cerebral vasoconstriction with a cerebral blood flow decrease. In humans, 5% CO2 inhalation raises cerebral blood flow by approximately 50%, and 7% CO2, by 100%.33 It is not the CO2 tension per se that is responsible for the flow changes but the accompanying shift in perivascular pH. Increases of arteriolar CO2 tension in the cerebrospinal fluid do not affect pial arteriolar caliber unless acidification of the milieu occurs.34 Other mediators, such as prostaglandins and nitric oxide, have been shown to modify cerebral blood flow responses to increased CO2 tension.35,36
Adenosine
Several published studies indicate that adenosine, a purinergic nucleoside, is one of the major regulators of the cerebral circulation.37 In general, administration of exogenous adenosine potently relaxes cerebral arterioles and arteries with a subsequent increase in cerebral blood flow.37 Adenosine concentrations are rapidly elevated in response to a number of stimuli known to increase cerebral blood flow, such as hypoxia, hypotension, seizures, and neuronal activation. Theophylline, an antagonist of adenosine receptors, blocks the hyperemia observed during cerebral activation, findings that implicate adenosine, which can be produced during adenosine triphosphate catabolism, in the coupling between brain metabolism and blood flow.38
Endothelial Regulation
One of the major mechanisms involved in the regulation of cerebrovascular tone implicates endothelial factors. Indeed, more than simply a physical barrier, endothelial cells play a critical role in the regulation of brain perfusion through the production and release of potent relaxing and contracting factors that regulate the tone of underlying vascular muscle in response to chemical and mechanical stimuli. These factors include nitric oxide, endothelium-derived hyperpolarizing factors, certain eicosanoids, and endothelin-18,39 (see Fig. 41-7). In this section, we discuss briefly the involvement of nitric oxide and eicosanoids.
Nitric Oxide
Under basal conditions, tonic release of nitric oxide is a significant regulator of resting cerebral blood flow. Accordingly, NOS inhibition constricts cerebral arteries both in vitro and in vivo and decreases cerebral blood flow.8,39 Nitric oxide generated by eNOS diffuses from the endothelium to the smooth muscle where it binds to and stimulates soluble guanylate cyclase resulting in relaxation of smooth muscle. Reductions of cerebral blood flow in response to N-nitro-L-arginine, a nonselective NOS inhibitor, are absent in mice that are deficient in expression of the gene for eNOS, suggesting that endothelium is the primary source of nitric oxide that influences basal tone.40 Nitric oxide has also been shown to play an essential role in cerebral vasodilatation induced by shear stress, acetylcholine, bradykinin, and other humoral agents.8
Eicosanoids
Eicosanoids are a diverse group of substances derived from arachidonic acid, which is metabolized via three general pathways that involve cyclooxygenases (COX), lipooxygenases, and cytochrome P450 monooxygenases.41 These various eicosanoids can either promote vasodilatation or vasoconstriction. The eicosanoids are synthesized in a variety of cellular types, including neurons, astrocytes, and endothelial cells. As discussed, certain eicosanoids, released by astrocytes, have been implicated in the hyperemia associated with neuronal activation. Among the various eicosanoids, those produced by the COX pathway, namely prostaglandins and thromboxanes, are the most extensively studied. Prostaglandin I2, prostaglandin E2, and prostaglandin D2 are vasodilators, whereas prostaglandin F2α and thromboxane A2 mediate vasoconstriction.41 In contrast to the complex effects of the prostaglandins on the cerebral circulation, the cyclooxygenase inhibitor, indomethacin, consistently decreases cerebral blood flow in basal conditions and in response to certain stimuli such as hypercapnia.35,42 Prostaglandin I2 and stable prostaglandin I2 analogs produce relaxation of cerebral arteries in vitro and cerebral arterioles in vivo8 (see Fig. 41-7). After synthesis, PGI2 diffuses to the smooth muscle, where it activates adenylate cyclase through G protein–coupled receptors, increasing cyclic adenosine monophosphate and protein kinase A activity. The activation of protein kinase A causes K+ channels to open and produces smooth muscle hyperpolarization.43,44 Under normal conditions, prostaglandin I2 is the primary prostaglandin produced by the endothelium and is thought to play an important role in regulation of blood flow in response to intraluminal pressure, shear stress, and humoral agents.41 However, the relative contributions of prostaglandin I2 and the other prostaglandins to cerebrovascular tone are not well known and may vary as a function of age, species, vessel size, and external stimulation. These prostaglandins also show complex interactions with other endothelium-derived factors such as nitric oxide.
Edvinsson L, Krause DN, editors. Cerebral Blood Flow and Metabolism. Philadelphia: Lippincott, Williams and Wilkins, 2002.
Faraci FM, Heistad DD. Regulation of the cerebral circulation: role of endothelium and potassium channels. Physiol Rev. 1998;78:53-97.
Iadecola C. Neurovascular regulation in the normal brain and in Alzheimer’s disease. Nat Rev Neurosci. 2004;5:347-360.
1 Liebeskind DS. Collateral circulation. Stroke. 2003;34:2279-2284.
2 Alpers BG, Berry RG, Paddison RM. Anatomical studies in the circle of Willis in normal brains. Arch Neurol Psychiatry. 1959;81:409-418.
3 Edvinsson L, MacKenzie ET. General and comparative anatomy of the cerebral circulation. In: Edvinsson L, Krause DN, editors. Cerebral Blood Flow and Metabolism. Philadelphia: Lippincott, Williams and Wilkins; 2002:3-29.
4 Capra NF, Kapp JP. Anatomic and physiologic aspects of the venous system. In: Wood JH, editor. Cerebral Blood Flow: Physiologic and Clinical Aspects. New York: McGraw-Hill Book Company; 1987:37-58.
5 Shamji MF, Maziak DE, Shamji FM, et al. Circulation of the spinal cord: an important consideration for thoracic surgeons. Ann Thorac Surg. 2003;176:315-321.
6 Dunning HS, Wolff HG. The relative vascularity of various parts of the central and peripheral nervous system of the cat and its relation to function. J Comp Neurol. 1937;67:433-450.
7 Sokoloff L, Reivich M, Kennedy C, et al. The (14C)-deoxyglucose method for measurement of local cerebral glucose utilisation: theory, procedure, and normal values in the conscious and anesthetised albino rat. J Neurochem. 1977;28:897-916.
8 Faraci FM, Heistad DD. Regulation of the cerebral circulation: role of endothelium and potassium channels. Physiol Rev. 1998;78:53-97.
9 Anderson CM, Nedergaard M. Astrocyte-mediated control of cerebral microcirculation. Trends Neurosci. 2003;26:340-344.
10 Koehler RC, Gebremedhin D, Harder DR. Role of astrocytes in cerebrovascular regulation. J Appl Physiol. 2006;100:307-317.
11 Shepro D, Morel NM. Pericyte physiology. FASEB J. 1993;7:1031-1038.
12 Edvinsson L, Hamel E. Perivascular nerves in brain vessels. In: Edvinsson L, Krause DN, editors. Cerebral Blood Flow and Metabolism. Philadelphia: Lippincott, Williams and Wilkins; 2002:43-70.
13 Miller JD, Bell BA. Cerebral blood flow variations with perfusion pressure and metabolism. In: Wood JH, editor. Cerebral Blood Flow: Physiologic and Clinical Aspects. New York: McGraw-Hill Book Company; 1987:119-130.
14 Paulson OB, Strandgaard S, Edvinsson L. Cerebral autoregulation. Cerebrovasc Brain Metab Rev. 1990;2:161-192.
15 Powers WJ. Cerebral hemodynamics in ischemic cerebrovascular disease. Ann Neurol. 1991;29:231-240.
16 Baron JC. Positron tomography in cerebral ischemia. A review. Neuroradiology. 1985;27:509-516.
17 Kontos HA, Wei EP, Navari RM, et al. Responses of cerebral arteries and arterioles to acute hypotension and Hypertension. Am J Physiol. 1978;234:H371-H383.
18 Morita Y, Hardebo JE, Bouskela E. Influence of cerebrovascular parasympathetic nerves on resting cerebral blood flow, spontaneous vasomotion, autoregulation, hypercapnic vasodilation and sympathetic vasoconstriction. J Auton Nerv Syst. 1994;49:S9-S14.
19 Chillon JM, Baumbach GL. Autoregulation: arterial and intracranial pressure. In: Edvinsson L, Krause DN, editors. Cerebral Blood Flow and Metabolism. Philadelphia: Lippincott, Williams and Wilkins; 2002:395-412.
20 Goadsby PJ, Edvinsson L. Neurovascular control of the cerebral circulation. In: Edvinsson L, Krause DN, editors. Cerebral Blood Flow and Metabolism. Philadelphia: Lippincott, Williams and Wilkins; 2002:172-190.
21 Dauphin F, MacKenzie ET. Cholinergic and vasoactive intestinal polypeptidergic innervation of the cerebral arteries. Pharmacol Ther. 1995;67:385-417.
22 Hamel E, Lacombe P. Acetylcholine. In: Edvinsson L, Krause DN, editors. Cerebral Blood Flow and Metabolism. Philadelphia: Lippincott, Williams and Wilkins; 2002:222-247.
23 Vaucher E, Borredon J, Bonvento G, et al. Autoradiographic evidence for flow-metabolism uncoupling during stimulation of the nucleus basalis of Meynert in the conscious rat. J Cereb Blood Flow Metab. 1997;17:686-694.
24 Waite JJ, Holschneider DP, Scremin OU. Selective immunotoxin-induced cholinergic deafferentation alters blood flow distribution in the cerebral cortex. Brain Res. 1999;818:1-11.
25 Iadecola C. Neurovascular regulation in the normal brain and in Alzheimer’s disease. Nat Rev Neurosci. 2004;5:347-360.
26 Niwa K, Araki E, Morham SG, et al. Cyclooxygenase 2 contributes to functional hyperemia in whisker-barrel cortex. J Neurosci. 2000;20:763-770.
27 Peng X, Carhuapoma JR, Bhardwaj A, et al. Suppression of cortical functional hyperemia to vibrissal stimulation in the rat by epoxygenase inhibitors. Am J Physiol. 2002;283:H2029-H2037.
28 Zonta M, Angulo MC, Gobbo S, et al. Neuron-to-astrocyte signaling is central to the dynamic control of brain microcirculation. Nat Neurosci. 2003;6:43-50.
29 Takano T, Tian GF, Peng W, et al. Astrocyte-mediated control of cerebral blood flow. Nat Neurosci. 2006;9:260-267.
30 Roy CS, Sherrington C. On the regulation of the blood supply of the brain. J Physiol. 1890;11:85-108.
31 Kuschinsky W, Wahl M, Bosse O, et al. Perivascular potassium and pH as determinants of local pial arterial diameter in cats. A microapplication study. Circ Res. 1972;31:240-247.
32 Nguyen TS, Winn HR, Janigro D. ATP-sensitive potassium channels may participate in the coupling of neuronal activity and cerebrovascular tone. Am J Physiol. 2000;278:H878-H885.
33 Kety SS, Schmidt CF. The effects of altered arterial tensions of carbon dioxide and oxygen on cerebral blood flow and cerebral oxygen consumption of normal young men. J Clin Invest. 1948;27:484-492.
34 Kontos HA, Raper AJ, Patterson JL. Analysis of vasoactivity of local pH, PCO2 and bicarbonate on pial vessels. Stroke. 1977;8:358-360.
35 Pickard JD, Mackenzie ET. Inhibition of prostaglandin synthesis and the response of baboon cerebral circulation to carbon dioxide. Nat New Biol. 1973;245:187-188.
36 Iadecola C. Does nitric oxide mediate the increases in cerebral blood flow elicited by hypercapnia? Proc Natl Acad Sci U S A. 1992;89:3913-3916.
37 O’Regan M. Adenosine and the regulation of cerebral blood flow. Neurol Res. 2005;27:175-181.
38 Ko KR, Ngai AC, Winn HR. Role of adenosine in regulation of regional cerebral blood flow in sensory cortex. Am J Physiol. 1990;259:H1703-H1708.
39 Andresen J, Shafi NI, Bryan RM. Endothelial influences on cerebrovascular tone. J Appl Physiol. 2006;100:318-327.
40 Ma J, Meng W, Ayata C, et al. L-NNA-sensitive regional cerebral blood flow augmentation during hypercapnia in type III NOS mutant mice. Am J Physiol. 1996;71:H1717-H1719.
41 Busija DW. Prostaglandins and other eicosanoids. In: Edvinsson L, Krause DN, editors. Cerebral Blood Flow and Metabolism. Philadelphia: Lippincott, Williams and Wilkins; 2002:325-338.
42 Schumann P, Touzani O, Young AR, et al. Effects of indomethacin on cerebral blood flow and oxygen metabolism: a positron emission tomographic investigation in the anaesthetized baboon. Neurosci Lett. 1996;220:137-141.
43 Parkington HC, Coleman HA, Tare M. Prostacyclin and endothelium-dependent hyperpolarization. Pharmacol Res. 2004;49:509-514.
44 Corriu C, Feletou M, Canet E, et al. Endothelium-derived factors and hyperpolarization of the carotid artery of the guinea-pig. Br J Pharmacol. 1996;119:959-964.