CHAPTER 130 Anatomy of the Vestibular System
The semicircular canals are arranged as a set of three orthogonal sensors (Fig. 130-1)—that is, each canal is at approximately right angles to the other two. Each canal is maximally sensitive to rotations that lie in the plane of that canal. The result of this arrangement is that the three canals can uniquely specify the direction and amplitude of any arbitrary head rotation. Each of the canals acts as an integrating accelerometer; the necessary stimulus for the canal is an angular acceleration, but the information that is encoded by the firing of the afferent nerve fiber is more closely related to angular velocity. Finally, the canals are organized into functional pairs wherein both members of the pair lie in the same plane. Any rotation in that plane is excitatory to one of the members of the pair and inhibitory to the other. Although in the horizontal system the two horizontal canals form a functional pair, the situation is more complex in the vertical system. Here, the anterior canal on one side is parallel and coplanar with the posterior canal on the opposite side; for example, the right anterior canal and the left posterior canal form a functional pair.
The vestibular system forms the basis for many fundamental reflexes: the vestibulocollic reflex (head stabilization), vestibulospinal reflex (control of upright posture) and vestibuloocular reflex (retinal image stabilization). The last of these, the vestibuloocular reflex, has been studied far more extensively than the others and is the best understood; this reflex forms the basis for most clinical testing (e.g., calorics, rotation tests). See also Chapters 163 and 164 for clinical applications.
Embryology of the Vestibular Apparatus
The development of the inner ear is a complex process that starts at the beginning of the 4th week and is completed at about 25 weeks of gestational age. By that time, the vestibular apparatus has achieved adult form and size. A brief description of this process of development in human embryos follows. Further details can be found by consulting more detailed references.1–3 An excellent description of the molecular aspects of development can be found in Post,4 and a review by Kelley5 discusses these molecules in relation to potential hair cell regeneration (see also Chapters 1 and 2).
When the human embryo reaches the seven-somite stage (about 22 days), surface ectoderm overlying the future site of the inner ear (at about the level of the first occipital somite) thickens to form the otic placode. The otic placode invaginates into the mesenchyme, forming an otic pit. At about 30 days, the otic pit becomes pinched off, forming the otic vesicle or otocyst (see Fig. 130-1). Concurrently at about 4 weeks, a portion of the neural crest migrates to the vicinity of the otic vesicle and becomes the acousticofacial ganglion. The geniculate ganglion soon migrates away from this cluster of neurons, leaving the vestibulocochlear ganglion in close proximity to the otic vesicle.
The saccular chamber differentiates by expansion and coiling of the cochlear duct. This duct becomes separated from the sacculus by a narrowing of the duct at its dorsal end to form the ductus reuniens. While morphogenesis proceeds within the otocyst, histogenesis of the sensory epithelium is also occurring. The arrival of afferent endings in the epithelium precedes hair cell differentiation.6,7 In the 3rd week, a common macula, or specialized neuroepithelium, appears. Its upper part becomes the utricular macula and crista ampullaris of the superior and lateral semicircular ducts, and its lower part becomes the saccular macula and crista ampullaris of the posterior semicircular duct. At 9 weeks, the hair cells in the vestibular end organs are well differentiated, and they exhibit typical synapses with nerve endings. The maculae reach adult form at about 14 to 16 weeks; the cristae, at about 23 weeks; and the organ of Corti, at about 25 weeks. The mesoderm surrounding the membranous labyrinth becomes the bony otic capsule, or bony labyrinth. The membranous labyrinth is suspended in fluid (perilymph) within the bony labyrinth by a loose connective tissue termed periotic tissue.
Overall Organization of Labyrinth: Relationship to Skull and Cochlea
The vestibular apparatus is enclosed within a bony labyrinth, the vestibule, in the petrous portion of the temporal bone. The vestibular end organs include three semicircular canals, each oriented in a different plane, and two maculae, one roughly in the horizontal plane (the utriculus) and one in the vertical plane (the sacculus). There are two vertical semicircular canals, the anterior (also known as the superior) and posterior canals, and one horizontal (also known as the lateral) canal. The vertical canals are oriented roughly at 45 degrees in relation to the sagittal plane, and the horizontal canal is tilted upward about 30 degrees anteriorly from the horizontal plane (Fig. 130-2). The five vestibular end organs, along with the cochlea, are contained within an endolymph-filled membranous labyrinth (the endolymphatic space), which is itself contained in the perilymph-filled bony labyrinth (the perilymphatic space) (Fig. 130-3).
The vestibule is situated between the internal auditory meatus anteromedially and the middle ear cavity laterally (Fig. 130-4). The entrance to the mastoid antrum (the aditus ad antrum) is just lateral to the horizontal semicircular canal. The cochlea sits anterior to the vestibule and is connected to the vestibule by the narrow ductus reuniens (Fig. 130-5; see Fig. 130-3). Posterior and lateral to the vestibule are the mastoid air cells. Directly medial is the posterior cranial fossa, into which the endolymphatic duct and sac extend beneath the dura.
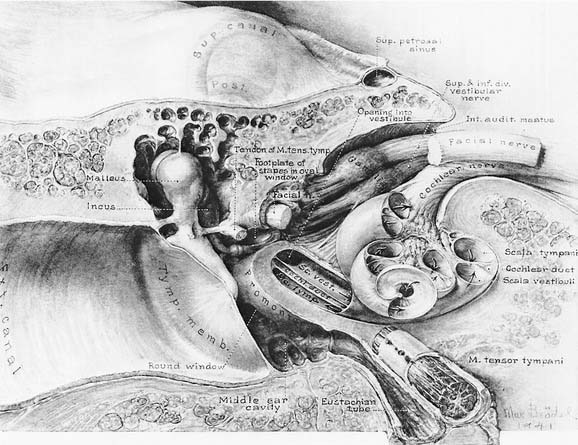
Figure 130-4. Course of vestibulocochlear nerve within the auditory meatus.
(From Brodel M. Three Unpublished Drawings of the Anatomy of the Human Ear. Philadelphia: Saunders; 1946.)
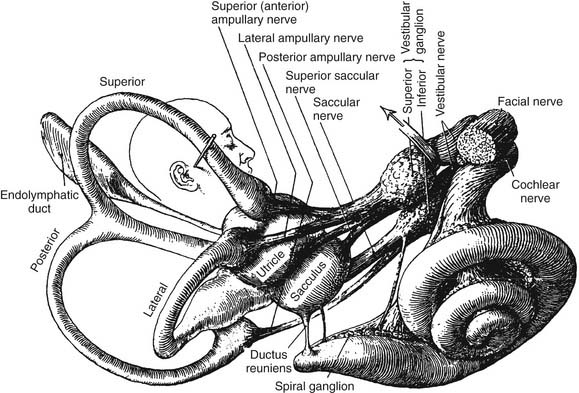
Figure 130-5. Distribution of vestibular nerve to end organs.
(From Brodel M. Three Unpublished Drawings of the Anatomy of the Human Ear. Philadelphia: Saunders; 1946.)
The seventh (facial and intermediate) and eighth (vestibulocochlear) cranial nerves emerge from the brainstem laterally at the cerebellopontine angle. They enter the vestibule and cochlea from the internal auditory meatus (see Fig. 130-4), located medial to a point midway between the cochlea and vestibule. The facial nerve lies anterior and dorsal to the vestibulocochlear nerve. The two nerves separate just inside the meatus, and the facial and intermediate nerves continue laterally in their own canal, past the vestibule. When past the vestibule, the facial nerve makes a 90-degree turn inferiorly to exit the temporal bone through the stylomastoid foramen. The vestibulocochlear nerve splits into a vestibular division, which turns posteriorly to supply the vestibule, and a cochlear division, which turns anteriorly to supply the cochlea.
The vestibular (Scarpa’s) ganglion sits at the bottom of the internal auditory meatus. It has two parts, the superior vestibular ganglion and the inferior vestibular ganglion (see Fig. 130-5). Large ganglion cells in the superior and inferior ganglia provide afferent innervation to the central regions of the cristae and maculae, and small ganglion cells innervate the peripheral regions of these end organs (the regional specialization within the end organs is discussed in more detail subsequently). A nerve branch is associated with each ganglion. The superior (or anterior) vestibular nerve supplies the anterior and horizontal cristae and the utricular macula. The inferior (or posterior) vestibular nerve supplies the posterior canal and saccular macula. There are also a few small branches that anastomose between the larger divisions of CN VIII. One such small branch is Voit’s anastomosis, which runs from the superior vestibular nerve to the anterosuperior part of the sacculus. Another is the vestibulocochlear (Oort’s) anastomosis, which runs from the inferior vestibular nerve to the cochlear nerve and carries cochlear efferents (see later). In addition, some intermediate nerve fibers cross to the vestibular nerve proximal to the superior vestibular ganglion, and others cross back to the intermediate nerve distal to Scarpa’s ganglion. It has been suggested that this facial-vestibular anastomosis carries parasympathetic innervation to the vestibular labyrinth; for details on these and other small fiber connections, see Lindemann.8
Efferent Innervation of Peripheral Vestibular Apparatus
The efferent supply to the vestibular end organs arises from a small group of about 200 neurons lateral to the abducens nucleus and the genu of the facial nerve, the so-called group e (see later).9 These neurons project ipsilaterally and contralaterally (Fig. 130-6). The contralateral pathway crosses the midline at the level of the facial genu and joins the ipsilateral pathway; both pass ventral to the vestibular nucleus. At this point, they are joined by cochlear efferents originating from the superior olivary complex (olivocochlear bundle). All of the efferents enter the vestibular nerve, coursing through the middle of the nerve in a small distinct bundle. At the end organs, these few fibers branch profusely to innervate the entire sensory epithelium. More recent work suggests that the ipsilaterally projecting efferents supply the central regions of the crista, whereas the contralaterally projecting efferents supply the peripheral zone.10 The efferent fibers terminate as highly vesiculated boutons, making synaptic contacts with hair cells and afferent fibers.11,12 For an excellent review of the neurochemistry of the vestibular efferent system, see Goldberg and colleagues.13
Autonomic Supply of Peripheral Vestibular Apparatus
Postganglionic sympathetic fibers from neurons in the superior cervical ganglion also innervate the vestibular end organs. They are of two types: perivascular and nonvascular. The nonvascular sympathetic fibers run among the myelinated afferent fibers. The terminals of these nonvascular sympathetic fibers are found as free endings near the cells of Scarpa’s ganglion, distal to the ganglion and underneath the sensory epithelium. They do not seem to penetrate the basement membrane into the sensory epithelium and do not seem to have a direct effect on the hair cells or afferent fibers.14 Their functional role remains unexplored, along with the functional role of any parasympathetic innervation of the vestibular sensory epithelium.
Blood Supply to Vestibular End Organs
The main blood supply to the vestibular end organs is through the internal auditory (labyrinthine) artery, which arises most often (45%) from the anterior cerebellar artery, superior cerebellar artery, or basilar artery, according to a radiographic study by Wende and coworkers.15 Shortly after entering the inner ear, the labyrinthine artery divides into two branches: the anterior vestibular artery and the common cochlear artery (Fig. 130-7). The anterior vestibular artery provides the blood supply to most of the utriculus and the superior and horizontal ampullae, and some blood to a small portion of the sacculus. The common cochlear artery forms two divisions, the proper cochlear (or spiral modiolar) artery and the vestibulocochlear artery. The latter divides into a cochlear ramus and a vestibular ramus, also known as the posterior vestibular artery. The posterior vestibular artery is the source of the blood supply to the posterior ampulla, the major part of the sacculus, and parts of the body of the utriculus and horizontal and superior ampullae.
Anatomy of End Organs
At the dilated end of each semicircular duct is the ampulla. It contains the neuroepithelium (crista ampullaris), the cupula, supporting cells, connective tissue, blood vessels, and nerve fibers. The crista is a saddle-shaped, raised section of the wall that extends across the floor of the ampulla at right angles to its long axis. The crista has been found to be divided into central (near the apex) and peripheral (on the slope) zones based on the morphology and physiology of vestibular afferents supplying the different regions (see later).16,17 The shape of the crista facilitates maximal packing of the specialized mechanoreceptor hair cells.
The hair cells and the supporting cells are modified columnar epithelial cells that have microvilli on their apical surfaces. In the hair cells, many of these microvilli are elongated to form stereocilia, which are grouped in an organ pipe–like arrangement (Fig. 130-8). In addition, each hair cell has a single long kinocilium, a true cilium showing the 9 + 2 arrangement of microtubules. This kinocilium is longer than the stereocilia and eccentrically located, imparting a certain polarization to the hair cell that has important functional implications (see Chapter 163). In the cristae, the kinocilium on each hair cell is located on one end of the cell (Fig. 130-9). In the horizontal cristae, the kinocilia are located on the side of the hair cell that is closest to the utriculus. In the vertical cristae, the kinocilia are located on the side of the hair cell furthest from the utriculus, the canalicular side. The entire hair bundle extends upward into the cupula (Fig. 130-10).
The cupula is a gelatinous mass, consisting of mucopolysaccharides within a keratin meshwork,18 which extends from the surface of the cristae to the roof and lateral walls of the membranous labyrinth, forming a fluid-tight partition. There is a distinct subcupular space in the region of the cupula overlying the apex of the center of the crista (see Fig. 130-10). This subcupular space is believed to provide space for freedom of movement and more sensitive responses to endolymph flow for the stereocilia on the hair cells in the central zone. The space may be artifactual and due to fixation methods commonly used to prepare tissue for histology. The specific gravity of the cupula is approximately 1, which is about the same as that of the endolymph.19 This matching of the specific gravity of the cupula and the endolymph is necessary to prevent the cupula from floating upward in certain head positions and causing an enduring nystagmus. Disruption of this match in specific gravity is likely the cause of postalcoholic nystagmus.
A torsion pendulum model is used to describe the mechanics of cupular and endolymph displacement.20 For a description of the mathematic details of the response of canal afferents, see Goldberg and Fernández.21 More details are also given in Chapter 163.
Similar to the cristae ampullares, the maculae of the utriculus and sacculus consist of neuroepithelium, supporting cells, blood vessels, and nerve fibers. The utricular macula is oriented in the horizontal plane, and the saccular macula is oriented in the vertical plane (Fig. 130-11). Cilia from the hair cells in both maculae extend upward into their respective otolithic membranes (Fig. 130-12)—gelatinous membranes analogous to the cupula. In the upper surface of the otolithic membranes, the otoliths (or otoconia) are embedded. Otoliths are inorganic crystalline deposits composed of calcium carbonate or calcite.22 They vary in size from 0.5 to 30 µm, with most being about 5 to 7 µm.8 The specific gravity of the otolithic membrane is much higher than that of the endolymph, about 2.71 to 2.94.19 Within the otolithic membrane is the striola,23 a specialized central region that has a snowdrift-like appearance. The striola is identifiable as a thin stripe running down the center of the otolith membranes of both maculae. In the striola, the otoliths are very small (about 1 µm), and the thickness of the otolithic membranes is either reduced, as in the utricular macula, or increased, as in the saccular macula. These regional differences in the otolithic membranes are paralleled by morphologic and physiologic differences in the afferent fibers supplying the hair cells in the underlying sensory epithelium.24–26
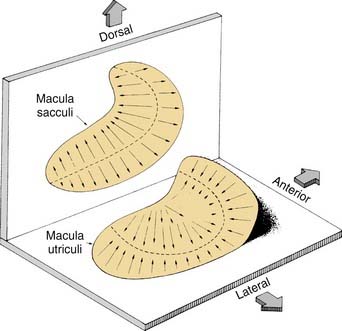
Figure 130-11. Orientation of two maculae. Utricular macula is in horizontal plane, and saccular macula is in vertical plane.
(From Barber HO, Stockwell CW. Manual of Electronystagmography. St. Louis: Mosby-Year Book; 1976.)
The kinocilia on the hair cells in the maculae are also dynamically polarized, but the pattern of polarization is much more complex than in the cristae (see Figs. 130-9 and 130-11). In the utricular macula, the kinocilia are oriented so that they point toward the striola, whereas in the saccular macula, the kinocilia point away from the striola. Because both maculae are curved areas, and the striolae are curved lines, the arrangement is so complex (see Fig. 130-11) that static head tilts in any direction cause some hair cells to be excited and others to be inhibited in one or both of the otolithic organs. The stimulus is encoded by means of stimulation of hair cells in the appropriate sector of the macula.
The otolith organs are sensitive not only to gravity, but also to other linear acceleration forces, such as forward motion and bobbing movements of the head during walking. Static head tilts are represented by a vector and the afferent response predicted by a trigonometric function based on the tilt angle. One would expect a linear model based on this simple relationship; however, there is a response asymmetry in that the excitatory response is larger than the inhibitory response. Consequently, the equation describing this force-response relationship is by necessity nonlinear (for more mathematical details, see Fernández and Goldberg27,28).
Cellular Morphology of Vestibular Sensory Epithelium
The sensory epithelium is composed of several different elements: hair cells, supporting cells, afferent nerve fibers and their synaptic terminals, and efferent nerve fibers and their synaptic boutons. Two basic cell types are present within the sensory epithelium: supporting cells and hair cells (Fig. 130-13). Supporting cells extend from the basement membrane to the apical surface. Their nuclei are usually found just above the basement membrane and below the hair cells. In sections taken tangential to the apical surface, several supporting cells can be seen to form a ring around an individual hair cell. The supporting cells themselves contain well-developed Golgi complexes, many mitochondria, and occasional lipid droplets. The upper part of the supporting cells contains large numbers of round or ovoid granules. These secretory granules are likely responsible for the formation of the cupula and otolithic membrane.29,30
Synaptic Morphology of Vestibular Afferents
Hair cells are of two types, type I and type II, defined by the presence or absence of a calyx, or chalice, a specialized type of large afferent ending that completely surrounds the cell except for the near-apical and apical surfaces (see Fig. 130-13). Type I hair cells are flask-shaped cells surrounded by a calyx ending. Usually, a calyx surrounds a single type I hair cell. In this case, the calyx ending is termed a simple calyx ending. Sometimes one calyx surrounds two to four type I hair cells (Fig. 130-14); this is termed a complex calyx ending. Complex calyx endings are much more common in the central zone (or striola) than in the periphery (or extrastriola). Histologically, the existence of complex endings is a criterion that can be used to define the central zone.
The ratio of type I to type II hair cells is approximately 1 : 1, and this ratio changes very little from the central to the peripheral zone, based on studies in which rodents have been used to quantify cell distributions.31,32 More recent work in the crista of the squirrel monkey has shown, however, that although the total number of hair cells is similar to rodents, the ratio of type I and type II hair cells in the central zone is increased from 1 : 1 to about 5 : 1 in primates compared with rodents.33 This ratio may reflect the relative importance of type I hair cells for primates. The same is not the case, however, for the monkey otolith organs, in which type II hair cells outnumber type I hair cells by 2 : 1.34
Type II hair cells are cylinder-shaped cells that are contacted at their basal surfaces by numerous afferent and efferent synaptic boutons. Afferent boutons contain mitochondria and few vesicles and receive synaptic contacts from the hair cell. They transmit the impulse centrally to the vestibular nuclei and are postsynaptic to the hair cell. Efferent boutons contain many vesicles and smaller mitochondria and vesicles than those found in afferent boutons. They form synapses with hair cells and afferents, and transmit impulses from the efferent group of neurons located in the brainstem. It has long been assumed that hair cells from different regions had approximately the same number of afferent boutons contacting them, and that each bouton formed about the same number of synapses with a given hair cell. More recent ultrastructural work12,34 has shown that there are regional variations in the synaptic innervation of type II hair cells (see Fig. 130-14). Type II hair cells in the periphery (or extrastriola) make synaptic contact with many afferent boutons, each of which receives one synapse from the adjacent hair cell. Type II hair cells in the central zone (or striola) make synaptic contact with relatively few afferent boutons, but make multiple contacts with each of these. In addition, type II hair cells in the central zone make synaptic contacts with the outer surface of the calyx endings surrounding type I hair cells, a type of synapse rarely found in the peripheral zone.
Synaptic Morphology and Function of Vestibular Afferents
More recent morphologic and physiologic work on vestibular afferents16,17,24–26,33,35 has defined, structurally and functionally, specialized subregions within the sensory epithelium (loosely analogous to the fovea and periphery of the retina): the central and peripheral zones of the vestibular sensory epithelium. These studies have involved experiments in which vestibular afferent nerve axons were impaled with microelectrodes; characterized electrophysiologically using responses to galvanic, rotational, and linear acceleration stimuli; and injected with horseradish peroxidase used as an intracellular dye. The horseradish peroxidase–filled afferent nerve fibers were recovered histologically, their position within the sensory epithelium was reconstructed, and their nerve terminals and axons were drawn. In this manner, these investigators were able to define morphologically three types of afferent endings—pure calyx (or chalice) endings, bouton endings, and dimorphic endings (which contained calyx and bouton terminals) (Figs. 130-15 and 130-16)—and to determine that the distribution of these three types was segregated within the sensory epithelium.
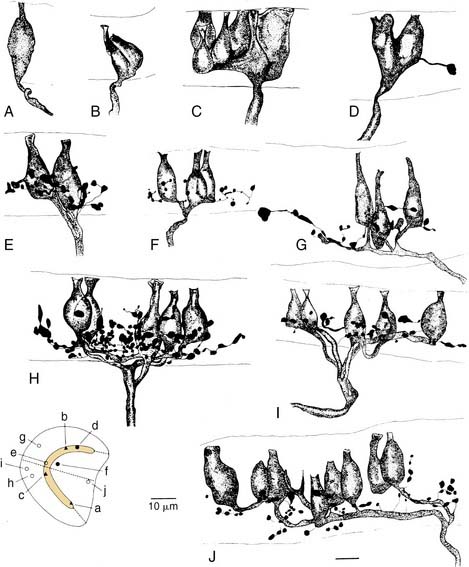
Figure 130-16. A-J, Reconstruction of 10 utricular afferents labeled by intra-axonal injections of horseradish peroxidase and their locations on a standard map of the macula (lower left). Bar, 10 µm. See Figure 130-15 for further explanation.
(From Goldberg JM, Desmadryl G, Baird RA, et al. The vestibular nerve of the chinchilla. V. Relation between afferent response properties and peripheral innervation patterns in the utricular macula. J Neurophysiol. 1990;63:791-804.)
These three morphologic types of afferents fell into two physiologic types that were also segregated within the sensory epithelium. Pure calyx endings had a very irregular discharge pattern and were insensitive (low gain) in response to sinusoidal head rotation. Calyx endings were found only in the central region. Bouton and dimorphic endings were distributed along a continuum in which discharge regularity and response dynamics were linearly correlated; that is, bouton endings were very regularly discharging and had low sensitivity to sinusoidal head rotation, whereas dimorphic endings could vary from very regular to very irregular in discharge rate, and from very low gain to very high gain response dynamics. Bouton endings were always found near the periphery. Dimorphic endings were found throughout the sensory epithelium, but irregular dimorphic endings were found in the central zone, and regular dimorphic endings were in the peripheral zone. The functional classification of vestibular afferents into regularly and irregularly discharging afferents, which correlates with numerous other physiologic and morphologic features,13,36 has proved useful in understanding peripheral and central terminations, as discussed subsequently.
Central Pathways of Vestibular System
Terminations of Vestibular Nerve in Brainstem
The vestibular nerve enters the brainstem at the ventrolateral aspect of the pontomedullary junction in close proximity to the cochlear and facial nerves, which accompany it to the internal auditory meatus. After entering the brainstem, the fibers of the vestibular nerve course dorsally and medially, passing between the inferior cerebellar peduncle and the descending tract of the trigeminal nerve, and enter the vestibular nuclei. On entering the nuclei, most vestibular fibers give rise to two branches in the vestibular nuclei, one rostral and one caudal (Fig. 130-17).37 The rostral branch projects to the superior and medial vestibular nuclei, and sends a collateral branch to the cerebellum. The caudal branch projects to the inferior and medial vestibular nuclei and to the ventrolateral part of the lateral vestibular nucleus. Regularly discharging vestibular afferents, described in the previous paragraph, terminate on small to medium-sized vestibular nuclear neurons, whereas irregularly discharging afferents terminate on medium-sized to large vestibular nuclear neurons (see Fig. 130-17).38 There are two major projection sites in the brain—the vestibular nuclei and the cerebellum.39 No primary vestibular afferents cross the midline.
Projections of Vestibular Nerve to Cerebellum
It has been estimated that about 70% of primary vestibular afferents40 terminate by means of mossy fibers in the granular layer of the most caudal lobules of the cerebellar vermis, specifically in the uvula and nodulus. This region of the cerebellum is thought to be involved in coordinating movements of the head and eyes. Purkinje cells in the posterior vermis project either to the fastigial nucleus or to the vestibular nuclei. The fastigial nucleus is the deep cerebellar nucleus that receives collateral projections from primary afferents in addition to inputs from the vermis.41,42 Another region of the cerebellar cortex that projects directly to the vestibular nuclei, the cerebellar flocculus, does not seem to receive significant direct inputs from the vestibular nerve, although it does receive secondary inputs.43
Anatomic Subdivisions of Vestibular Nuclei
The vestibular nuclei have been classically divided into four main regional subdivisions44–46—superior, medial, lateral, and inferior vestibular nuclei—and several minor cell groups (Fig. 130-18). The superior vestibular nucleus (of Bechterew) is located dorsally and rostrally in the vestibular complex. It can be subdivided into a central region that contains many medium-sized neurons and a peripheral region that contains mostly smaller cells. The superior vestibular nucleus is involved in vestibuloocular reflex pathways. Neurons in this nucleus fire in relation to eye movements and head movements (see Chapter 163), and a prominent efferent projection of this nucleus is to the oculomotor nucleus via the medial longitudinal fasciculus. This tract is particularly important in the control of the vestibuloocular reflex.
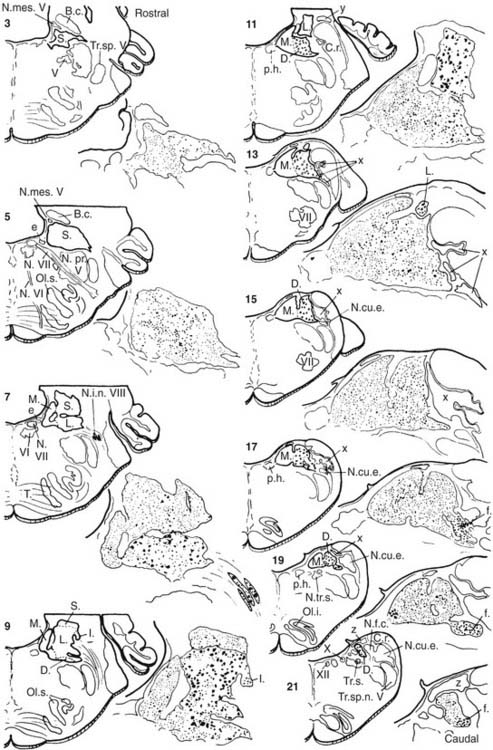
Figure 130-18. Different subdivisions of vestibular nuclei in the cat showing their relationship to other brainstem structures. The cells in each section are also pictured at higher magnification to illustrate the varying size of neurons in different subdivisions. The cell group e from the work of Goldberg and Fernández9 has been added to this figure. B.c., brachium conjunctivum; C.r., restiform body; D., descending (inferior) vestibular nucleus; e, small cell group containing vestibular efferent neurons; f., ventrolateral caudal subdivision of the descending vestibular nucleus; l., subdivision of the descending vestibular nucleus containing medium-sized cells; L., lateral vestibular nucleus; M., medial vestibular nucleus; N.cu.e., external cuneate nucleus; N.f.c., nucleus fasciculus cuneatus; N.i.n.VIII, interstitial nucleus of the vestibular nerve; N.mes.V, mesencephalic nucleus of trigeminal nerve; N.pr.V, principal nucleus of trigeminal nerve; N.tr.s., nucleus of tractus solitarius; N.VI and N.VII, abducens nerve and facial nerve; Ol.s., superior olive; Ol.i., inferior olive; p.h., nucleus prepositus hypoglossi; S., superior vestibular nucleus; T., trapezoid body; Tr.s., tractus solitarius; Tr.sp.V, spinal tract of trigeminal nerve; Tr.sp.n.V, tract of spinal trigeminal nucleus; V, trigeminal motor nucleus; VI, abducens nucleus; X, dorsal motor nucleus of the vagus nerve; XII, hypoglossal nucleus; x, y and z, cell groups x, y, and z.
(Slightly modified from Brodal A, Pompeiano O, Walberg H. The Vestibular Nuclei and Their Connections, Anatomy and Functional Correlations. Edinburgh, Scotland: Oliver & Boyd; 1962.)
The lateral vestibular nucleus (of Deiters) can be subdivided on anatomic and functional grounds into two subnuclei: the dorsal lateral vestibular nucleus (or Deiters’ nucleus) and the ventral lateral vestibular nucleus. The dorsal lateral vestibular nucleus contains the many giant cells of Deiters that give rise to the lateral vestibulospinal tract, and the ventral lateral vestibular nucleus contains medium-sized neurons that give rise to vestibuloocular pathways, the medial vestibulospinal tract, and vestibulothalamic pathways. The two subdivisions of the lateral vestibular nucleus also differ in regard to their afferent inputs (see later). There is a somatotopic representation within the lateral vestibular nucleus,47 such that the anterior and superior part of the nucleus supplies the cervical cord, whereas the posterior and inferior part supplies the lumbosacral cord. The lateral vestibulospinal tract terminates directly on the ventral horn cells either directly or indirectly by means of interneurons. This tract is particularly important for vestibulospinal and vestibulocollic reflexes.
The medial vestibular nucleus (of Schwalbe) extends rostrocaudally in the brainstem from the level of the abducens nucleus to the hypoglossal nucleus. It is bounded medially by a functionally related nucleus, the nucleus prepositus hypoglossi, and laterally by the inferior vestibular nucleus. More recent studies indicate that the medial vestibular nucleus can be subdivided into a rostral magnocellular region and a parvocellular region and a caudal region with small to medium-sized neurons.39,48 The rostral medial vestibular nucleus, similar to the superior nucleus, contains many neurons that project to the oculomotor nuclei and whose firing behavior is related to eye movements.49–51 Little is known about the functions of the caudal region of the medial vestibular nucleus, although many of the cells in this region seem to project to the cerebellum.52 The medial vestibular nucleus also gives rise to the medial vestibulospinal tract, which descends in the medial longitudinal fasciculus to terminate on interneurons in the cervical spinal cord, and ascends to terminate in the eye motor nuclei. This tract is particularly important for cervicovestibuloocular reflexes.
The inferior (or descending) vestibular nucleus merges rostrally with the ventral lateral vestibular nucleus and extends most caudally in the vestibular complex. This region of the vestibular nuclei is one of the primary recipients of vestibular afferents that innervate the otolithic organs.53 Some of the cells in this nucleus contribute to the vestibulospinal pathways, but the major projections from this area seem to be to the cerebellum and reticular formation.
The minor cell groups associated with the vestibular nuclei have been named alphabetically in lower case letters: cell groups f, l, x, y, z, and e. Cell group f is a caudal subdivision of the descending vestibular nucleus, consisting of medium-sized cells. Cell group l is a small group of medium-sized cells at the caudal and lateral border of the lateral vestibular nucleus, adjacent to the restiform body. It can be distinguished from the many giant cells of Deiters in the nucleus. In addition, most of its projections are to the spinal cord below T1.47 Cell group z is not properly included in the vestibular nuclei. It is located in the caudal part of the vestibular complex, and it receives its primary input from fibers that travel in the spinal dorsal columns; its cells contribute to ascending somatosensory pathways to the thalamus.
Cell groups x and y are important functional subdivisions of the vestibular nuclei. Cell group x is also located in the caudal part of the vestibular complex. This area receives a prominent input from the spinal cord, and it projects to the cerebellum. The y group is located caudal and lateral to the superior vestibular nucleus and has a dorsal boundary with the lateral vestibular nucleus and a ventral boundary with the inferior cerebellar peduncle. The dorsal and ventral parts of the y group of cells seem to be functionally different. The ventral part of the y group consists of small fusiform neurons that receive inputs from vestibular afferents that innervate the sacculus.53 The dorsal part of the y group contains larger neurons and is traversed by a fiber tract, termed the floccular peduncle, which passes from the cerebellar flocculus to the vestibular nuclei. Many of the cells in the dorsal part of the y group seem to be functionally related to the flocculus and to eye movement control, particularly eye movements in the vertical plane.54
Cell group e (also see Fig. 130-6) is associated with the vestibular nuclei, but contains neurons projecting out to the labyrinth instead of receiving primary afferents. There is some suggestion, however, that it may receive collaterals of primary afferents, which would constitute a type of feedback circuit. It is located at the ventromedial aspect of the rostral medial vestibular nucleus, and it contains the cells that give rise to the efferent pathway from the brain to the vestibular end organs.9
Organization of Vestibular Nerve Inputs to Vestibular Nuclei
The vestibular nerve terminates in virtually all parts of the vestibular nuclei.53 The details of the terminations of the afferents that innervate individual vestibular end organs are not yet completely known, but cells whose activity is related primarily to otolith function have been found mainly in the dorsal lateral and inferior vestibular nuclei. In contrast, cells whose activity is primarily related to semicircular canal function have been found mainly in the superior, medial, and ventral lateral vestibular nuclei. Although the results of physiologic studies suggest that afferents that innervate different vestibular end organs do not synapse directly on the same cells in the vestibular nuclei,55 the activity of individual cells in the vestibular nuclei is not always clearly related to a single end organ.56 This finding suggests that there is some functional convergence of inputs from different end organs in some vestibular nuclear cells.
Some neurons in the vestibular nuclei do not receive direct inputs from the vestibular nerve. One example is a group of cells that respond preferentially to stimulation of the contralateral vestibular nerve (see later).57,58 The head movement sensitivity of most of the cells in the vestibular nuclei depends on bilateral activation of the vestibular end organs. Removal of one vestibular nerve typically reduces the head movement sensitivity of vestibular nuclear neurons approximately in half,59 which suggests that commissural pathways between the vestibular nuclei contribute significantly to the physiologic sensitivity of vestibular nuclear neurons.
Four physiologic types of neurons are found in the vestibular nuclei.57,58 These are described briefly here. Type I neurons are excited, whereas type II neurons are inhibited, by ipsilateral rotations of the head. The former receive ipsilateral input from primary afferents, whereas the latter receive either contralateral or commissural input. Type III neurons are excited by both directions of rotation, whereas type IV neurons are inhibited by either type of rotational acceleration. Type I neurons are the most common; there are also numerous type II neurons. Type III and type IV neurons are rare. Figure 130-19 shows a vestibular type I neuron in the medial vestibular nucleus that has been characterized morphologically and physiologically and projects to the abducens nucleus and other targets.60
Vestibular commissural pathways arise from every part of the vestibular nuclei except the dorsal lateral vestibular nucleus.48,61 Although there is a tendency for a particular region of the vestibular nuclei to project heavily to its contralateral counterpart, there is also considerable divergence in the commissural pathways to other parts of the vestibular nuclei (Fig. 130-20). Functionally, the commissural pathways between the vestibular nuclei seem to play an essential role in the ability to compensate for unilateral vestibular lesions.62 Because most vestibular nuclear neurons fire spontaneously even when the head is not moving,49 and because most vestibular functions are organized bilaterally, an imbalance between the spontaneous firing rate of one vestibular nucleus compared with the other results in the central perception of head movement and dysfunctional operation of reflex pathways (e.g., ocular nystagmus is usually produced when the vestibular nuclei are unilaterally damaged). The vestibular commissural pathways seem to play an important role in maintaining the balance in the output of the two sides.
Other Inputs to Vestibular Nuclei
The vestibular nerve is not the only source of input to the vestibular nuclei. Many regions of the brainstem and the cerebellum also project to the vestibular nuclei. Most of the nonvestibular inputs arise from the flocculonodular lobe of the cerebellum,63 from the fastigial nucleus,64 and from regions of the brainstem in the vicinity of the vestibular nuclei, although the vestibular nuclei also receive inputs from the spinal cord and from nuclei located in the midbrain and caudal diencephalon.
Although the function of each source of nonvestibular afferents to the vestibular nuclei is not completely known, the physiologic responses of vestibular nuclear neurons have been clearly shown to be affected by behaviors and stimuli not related to vestibular nerve activity. Perhaps the most common nonvestibular input to the vestibular nuclei is an input from the visual system. Most vestibular nuclear neurons that are sensitive to head movements are also sensitive to movements of the visual world; that is, they are sensitive to optokinetic stimuli.65 The semicircular canals are insensitive to low-frequency (<0.05 Hz) movements of the head. The visual system is capable of detecting extremely low-frequency movements of the visual scene, however. The brain uses visual information to supplement the information obtained from the labyrinth. The anatomic pathways by which the visual optokinetic signals reach the vestibular nuclei have not been completely worked out, but several midbrain pretectal nuclei (in particular, the nucleus of the optic tract and the accessory optic nuclei) seem to play an essential role. These midbrain nuclei do not project directly to the vestibular nuclei themselves; rather, they project to other brainstem nuclei. These other nuclei project to the vestibular nuclei (e.g., the prepositus nucleus66) or to regions of the cerebellum that project to the vestibular nuclei (e.g., visual signals reach the cerebellum via the inferior olive67 and the pontine nuclei68).
Pathways from the cerebellum to the vestibular nuclei not only contribute to the visual responsiveness of vestibular nuclear neurons, but also are essential for adaptive modification of the head movement sensitivity of vestibuloocular pathways and compensation for unilateral vestibular lesions. The cerebellar flocculus is particularly important for adaptive modification of vestibuloocular reflex pathways.49,69 The Purkinje cells in this region of the cerebellar cortex are sensitive to optokinetic visual stimuli and to movements of small visual targets on the fovea.70,71 When eye movements produced by vestibuloocular pathways fail to stabilize images on the retina, the Purkinje cells of the flocculus change their firing rate and modify the head movement sensitivity of some of the vestibular nuclear neurons that project to the extraocular motor nuclei.
As noted earlier, many of the neurons in the superior, medial, and ventral lateral vestibular nuclei change their firing rate during eye movements even when the head is not moving and the vestibular nerve activity is unchanging. In a study by Chen-Huang and McCrea,72 the size of the utricular signal traveling to the oculomotor nucleus in the ascending tract of Deiters depended on the fixation distance. This finding implies the existence of an important neural multiplier system in the vestibular nuclei, and not a simple disynaptic relay between the utricle and the oculomotor nucleus. Vestibular nuclear neurons that project to the extraocular motor nuclei fire during steady fixation, saccadic eye movements, and ocular smooth pursuit.73,74 The vestibular nuclei apparently contribute to the generation of each of these oculomotor behaviors. The regions of the brain that provide these oculomotor-related inputs include the prepositus nucleus,75 the interstitial nucleus of Cajal,76 and the pontine and medullary reticular formation.77
The spinal cord, particularly the cervical spinal cord, is another important source of nonvestibular afferents to the vestibular nuclei. The neurons in the dorsal lateral vestibular nucleus that project to the spinal cord also receive inputs from the spinal segments to which they project.78 Neurons in the medial and ventral lateral vestibular nuclei that project to the extraocular motor nuclei receive inputs from the cervical spinal cord.79 These inputs are probably important for mediating the cervical ocular reflex, which is a reflex that can help the vestibuloocular reflex to stabilize gaze during head movements, particularly when the labyrinth is damaged. These pathways provide a possible explanation for the nystagmus often seen after neck injury.
There are other nonvestibular inputs that are functionally important but whose anatomy is poorly defined. It is likely that some vestibular nuclear neurons receive inputs related to central autonomic functions and behavioral state (e.g., alertness). Several observers have noted that the spontaneous firing rate and sensitivity to eye and head movements of many vestibular nuclear neurons are profoundly reduced during slow wave sleep or reduced alertness despite the fact that vestibular nerve activity is apparently unaffected. The central anatomic pathways that mediate these effects are unknown. The major afferent connections to the vestibular nuclei are summarized in Figure 130-21.
Efferent Projection Pathways from the Vestibular Nuclei
The vestibular nuclei project to numerous regions of the brainstem, cerebellum, and spinal cord. In addition, the vestibular nuclei are highly interconnected. The intrinsic connections of the vestibular nuclei are not limited to the commissural pathways described earlier, but also include connections between the various vestibular nuclei on the same side of the brain.48,61
Figure 130-22 provides an overview of the major vestibular efferent projections. All of the vestibular nuclei except the dorsal lateral vestibular nucleus contain neurons that project to the cerebellum. The cerebellar vermis, the flocculonodular lobe, and the fastigial nucleus are the regions of the cerebellum to which these vestibulocerebellar pathways project.80 These are the regions of the cerebellum that are thought to be involved in coordinating movements of the axial musculature, the head, and the eyes. The output of the cerebellar cortex is organized in parasagittal efferent strips.81 There is an efferent parasagittal cortical strip in the vermis that projects to the neurons in the dorsal lateral vestibular nucleus that gives rise to the lateral vestibulospinal tract. Another efferent strip in the vermis projects to the fastigial nucleus. Strips in the cerebellar cortex of the flocculonodular lobe project to the neurons in the superior, medial, and lateral vestibular nuclei that give rise to the vestibuloocular reflex pathways.69 There are reciprocal connections between the vestibular nuclei and regions in the cerebellum that receive vestibular inputs.
The medial, superior, and ventral lateral vestibular nuclei give rise to major bilateral efferent projections to the regions of the brainstem involved in controlling eye movements.82–84 The vestibular nuclei give rise to an important set of reflex pathways involved in the vestibuloocular reflex. The axons that make up these pathways usually join the medial longitudinal fasciculus near the abducens nucleus.
The function of the vestibuloocular reflex is to stabilize vision during head movements. This stabilization is accomplished by moving the eyes at the same speed as the head, but in the opposite direction. The vestibuloocular reflex pathways are organized reciprocally such that the motor neurons that innervate each extraocular muscle receive excitatory and inhibitory inputs from the vestibular nuclei. For more details on vestibuloocular reflex function, see Chapter 163.
A second major efferent vestibular pathway that aids in gaze stabilization is the medial vestibulospinal tract. This pathway originates from cells in the medial and ventral lateral vestibular nuclei and projects bilaterally in the medial longitudinal fasciculus to the ventral horn of the cervical spinal cord. Approximately half of these cells also contribute to the vestibuloocular reflex pathways via axon collaterals.85 The medial vestibulospinal tract functions to stabilize the head on the shoulders by generating neck muscle contractions that resist passive movements of the head.86
Vestibulothalamic pathways arise from cells in the ventral lateral vestibular nucleus and project to thalamic regions that border and include the ventral posterolateral, ventral posteromedial, and ventral lateral nuclei of the thalamus.87–89 The vestibular nuclei also project to another thalamic nucleus, the ventral lateral geniculate nucleus.90 This nucleus is adjacent to the dorsal lateral geniculate nucleus, and has anatomic connections that suggest it is involved in visual processing. Presumably, vestibulothalamocortical pathways play a role in the conscious perception of vertigo, or of self-motion, in the absence of visual stimulation.
Brodal A, Pompeiano O, Walberg H. The Vestibular Nuclei and Their Connections, Anatomy and Functional Correlations. Edinburgh, Scotland: Oliver & Boyd; 1962.
Büttner-Ennever JA, Gerrits NM, Mai JK. Vestibular system. In: Paxinos G, editor. The Human Nervous System. New York: Oxford University Press; 2004:1212-1240.
Desai SS, Zeh C, Lysakowski A. Comparative morphology of rodent vestibular periphery. I. Saccular and utricular maculae. II. Cristae ampullares. J Neurophysiol. 2005;93:251-266. 267-280
Goldberg JM, Brichta AM, Wackym PA. Efferent vestibular system: anatomy, physiology and neurochemistry. In: Anderson JH, Beitz AJ, editors. Neurochemistry of the Vestibular System. Boca Raton, FL: CRC Press; 2000:61-94.
Goldberg JM, Fernández C. Efferent vestibular system in the squirrel monkey: anatomical location and influence on afferent activity. J Neurophysiol. 1980;43:986-1025.
Highstein SM, Holstein GR. The anatomy of the vestibular nuclei. Prog Brain Res. 2006;151:157-203.
Horowitz SS, Blanchard JH, Morin LP. Intergeniculate leaflet and ventral lateral geniculate nucleus afferent connections: an anatomical substrate for functional input from the vestibulo-visuomotor system. J Comp Neurol. 2004;474:227-245.
Hughes I, Thalmann I, Thalmann R, et al. Mixing model systems: using zebrafish and mouse inner ear mutants and other organ systems to unravel the mystery of otoconial development. Brain Res. 2006;1091:58-74.
Kelley MW. Regulation of cell fate in the sensory epithelium of the inner ear. Nat Rev Neurosci. 2006;7:837-849.
King WM, Fuchs AF, Berthoz A. Neuronal activity in the mesencephalon related to vertical eye movements. In: Baker R, editor. Control of Gaze by Brain Stem Neurons, Vol 1. Developments in Neuroscience, Amsterdam: Elsevier; 1977:319-326.
Kotchabhakdi N, Rinvik E, Walberg F, et al. The vestibulothalamic projections in the cat studied by retrograde axonal-transport of horseradish-peroxidase. Exp Brain Res. 1980;40:405-418.
Lindemann HH. Studies on the morphology of the sensory regions of the vestibular apparatus. Ergeb Anat Entwicklungsgesch. 1969;42:1-113.
Lundberg YW, Zhao X, Yamoah EN. Assembly of the otoconia complex to the macular sensory epithelium of the vestibule. Brain Res. 2006;1091:47-57.
Lysakowski A, Goldberg JM. Morphophysiology of the vestibular sensory periphery. In: Highstein SM, Fay RR, Popper AN, editors. The Vestibular System. New York: Springer-Verlag; 2004:57-152.
Lysakowski A, Goldberg JM. A regional ultrastructural analysis of the cellular and synaptic architecture in the chinchilla cristae ampullares. J Comp Neurol. 1997;389:419-443.
McCrea RA, Baker R. Anatomical connections of the nucleus prepositus of the cat. J Comp Neurol. 1985;237:377-407.
McCrea RA, Strassman A, Highstein SM. Anatomical and physiological characteristics of vestibular neurons mediating the vertical vestibulo-ocular reflex of the squirrel monkey. J Comp Neurol. 1987;264:571-594.
Money KE, Bonen L, Beatty JD, et al. Physical properties of fluids and structures of vestibular apparatus of the pigeon. Am J Physiol. 1971;220:140-147.
Newlands SD, Perachio AA. Central projections of the vestibular nerve: a review and single fiber study in the Mongolian gerbil. Brain Res Bull. 2003;60:475-495.
Pompeiano O, Mergner T, Corvaja N. Commissural, perihypoglossal and reticular afferent projections to the vestibular nuclei in the cat: an experimental anatomical study with the method of retrograde transport of horseradish peroxidase. Arch Ital Biol. 1978;116:130-172.
Post JC. Molecular biology in pediatric otolaryngology. In: Bluestone CD, Stool SE, Alper CM, et al, editors. Pediatric Otolaryngology. Philadelphia: Saunders; 2003:66-81.
Sato F, Sasaki H. Morphological correlations between spontaneously discharging primary vestibular afferents and vestibular nucleus neurons in cat. J Comp Neurol. 1993;333:554-566.
Shimazu H, Precht W. Inhibition of central vestibular neurons from the contralateral labyrinth and its mediating pathway. J Neurophysiol. 1966;29:467-492.
Voogd J, Glickstein M. The anatomy of the cerebellum. Trends Neurosci. 1998;21:370-375.
Wersäll J, Bagger-Sjöback D. Morphology of the vestibular sense organ. In: Kornhuber HH, editor. Handbook of Sensory Physiology, Vestibular System, pt. 1: Basic Mechanisms. New York: Springer-Verlag; 1974:124-170.
1. Anson BJ, Davies J, Duckert LG. Developmental anatomy of the ear. In: Paparella MM, Shumrick DA, Gluckman JL, Meyerhoff WL, editors. Otolaryngology. Philadelphia: Saunders; 1991:3-21. 3rd ed
2. O’Rahilly R. The early development of the otic vesicle in staged human embryos. J Embryol Exp Morphol. 1963;11:741-755.
3. Tsai A-H, Stool SE, Post JC. Phylogenetic aspects and embryology. In: Bluestone CD, Stool SE, Alper CM, et al, editors. Pediatric Otolaryngology. Philadelphia: Saunders; 2003:1-21.
4. Post JC. Molecular biology in pediatric otolaryngology. In: Bluestone CD, Stool SE, Alper CM, et al, editors. Pediatric Otolaryngology. Philadelphia: Saunders; 2003:66-81.
5. Kelley MW. Regulation of cell fate in the sensory epithelium of the inner ear. Nat Rev Neurosci. 2006;7:837-849.
6. Sans A, Deschesne CJ. Afferent nerve ending development and synaptogenesis in the vestibular epithelium of human fetuses. Hear Res. 1987;28:65-72.
7. Sans A, Deschesne CJ. Early development of vestibular receptors in human embryos: an electron microscopic study. Acta Otolaryngol (Stockh) Suppl. 1985;423:51-58.
8. Lindemann HH. Studies on the morphology of the sensory regions of the vestibular apparatus. Ergeb Anat Entwicklungsgesch. 1969;42:1-113.
9. Goldberg JM, Fernández C. Efferent vestibular system in the squirrel monkey: anatomical location and influence on afferent activity. J Neurophysiol. 1980;43:986-1025.
10. Purcell IM, Perachio AA. Three-dimensional analysis of vestibular efferent neurons innervating semicircular canals of the gerbil. J Neurophysiol. 1997;78:3234-3248.
11. Engstrom H, Bergstrom B, Ades HW. Macula utriculi and macula sacculi in the squirrel monkey. Acta Otolaryngol (Stockh) Suppl. 1972;301:75-126.
12. Lysakowski A, Goldberg JM. A regional ultrastructural analysis of the cellular and synaptic architecture in the chinchilla cristae ampullares. J Comp Neurol. 1997;389:419-443.
13. Goldberg JM, Brichta AM, Wackym PA. Efferent vestibular system: anatomy, physiology and neurochemistry. In: Anderson JH, Beitz AJ, editors. Neurochemistry of the Vestibular System. Boca Raton, FL: CRC Press; 2000:61-94.
14. Hozawa K, Kimura RS. Vestibular sympathetic nervous system in guinea pig. Acta Otolaryngol (Stockh). 1989;107:171-181.
15. Wende S, Nakayama N, Schwerdtfeger P. The internal auditory artery (embryology, anatomy, angiography, pathology). J Neurol. 1975;210:21-31.
16. Baird RA, Desmadryl G, Fernández C, et al. The vestibular nerve of the chinchilla. II. Relation between afferent response properties and peripheral innervation patterns in the semicircular canals. J Neurophysiol. 1988;60:182-203.
17. Fernández C, Baird RA, Goldberg JM. The vestibular nerve of the chinchilla. I. Peripheral innervation patterns in the horizontal and superior semicircular canals. J Neurophysiol. 1988;60:167-181.
18. Dohlmann GF. The attachment of the cupulae, otolith and tectorial membranes to the sensory cell areas. Acta Otolaryngol (Stockh). 1971;71:89-105.
19. Money KE, Bonen L, Beatty JD, et al. Physical properties of fluids and structures of vestibular apparatus of the pigeon. Am J Physiol. 1971;220:140-147.
20. Steinhausen W. Uber die Beobachtung der Cupula in den Bogengansampullen des Labyrinths des lebenden Hechts. Pflügers Arch. 1933;232:500-512.
21. Goldberg JM, Fernández C. The vestibular system. In: Darian-Smith I, editor. Handbook of Physiology. The Nervous System, Vol III. Bethesda, MD: American Physiological Society; 1984:977-1031. Pt 2 Sect I
22. Ross MD, Pote KG, Perini F. Analytical studies of the organic material of otoconial complexes, including its amino acid and carbonate composition. In: Drescher DG, editor. Auditory Biochemistry. Springfield, IL: Charles C Thomas; 1985:500-514.
23. Werner CF. Die Differenzierung der Maculae im Labyrinth, insbesondere bei Säugetieren. Z Anat Entwickl-Gesch. 1933;99:696-709.
24. Fernández C, Baird RA, Goldberg JM. The vestibular nerve of the chinchilla. III. Peripheral innvervation patterns in the utricular macula. J Neurophysiol. 1990;63:767-780.
25. Goldberg JM, Desmadryl G, Baird RA, et al. The vestibular nerve of the chinchilla. IV. Discharge properties of utricular afferents. J Neurophysiol. 1990;63:781-790.
26. Goldberg JM, Desmadryl G, Baird RA, et al. The vestibular nerve of the chinchilla. V. Relation between afferent response properties and peripheral innervation patterns in the utricular macula. J Neurophysiol. 1990;63:791-804.
27. Fernández C, Goldberg JM. Physiology of peripheral neurons innervating otolith organs of the squirrel monkey. I. Response to static tilts and to long-duration centrifugal force. J Neurophysiol. 1976;39:970-984.
28. Fernández C, Goldberg JM. Physiology of peripheral neurons innervating otolith organs of the squirrel monkey. II. Directional selectivity and force-response relations. J Neurophysiol. 1976;39:970-984.
29. Hughes I, Thalmann I, Thalmann R, et al. Mixing model systems: using zebrafish and mouse inner ear mutants and other organ systems to unravel the mystery of otoconial development. Brain Res. 2006;1091:58-74.
30. Lundberg YW, Zhao X, Yamoah EN. Assembly of the otoconia complex to the macular sensory epithelium of the vestibule. Brain Res. 2006;1091:47-57.
31. Desai SS, Ali H, Lysakowski A. Comparative morphology of rodent vestibular periphery. II. Cristae ampullares. J Neurophysiol. 2005;93:267-280.
32. Desai SS, Zeh C, Lysakowski A. Comparative morphology of rodent vestibular periphery. I. Saccular and utricular maculae. J Neurophysiol. 2005;93:251-266.
33. Fernández C, Lysakowski A, Goldberg JM. Hair cell counts and afferent innervation patterns in the cristae ampullares of the squirrel monkey with a comparison to the chinchilla. J Neurophysiol. 1995;73:1253-1269.
34. Lysakowski A, Goldberg JM. Ultrastructural analysis of the cristae ampullares in the squirrel monkey (Saimiri sciureus). J Comp Neurol. 2008;511:47-64.
35. Lysakowski A, Minor LB, Fernandez C, et al. Physiological identification of morphologically distinct classes innervating the cristae ampullares of the squirrel monkey. J Neurophysiol. 1995;73:1270-1281.
36. Lysakowski A, Goldberg JM. Morphophysiology of the vestibular sensory periphery. In: Highstein SM, Fay RR, Popper AN, editors. The Vestibular System. New York: Springer-Verlag; 2004:57-152.
37. Lorente de No R. Anatomy of the eighth nerve: the central projection of the nerve endings of the internal ear. Laryngoscope. 1933;43:1-38.
38. Sato F, Sasaki H. Morphological correlations between spontaneously discharging primary vestibular afferents and vestibular nucleus neurons in the cat. J Comp Neurol. 1993;333:554-566.
39. Büttner-Ennever JA, Gerrits NM, Mai JK. Vestibular system. In: Paxinos G, editor. The Human Nervous System. New York: Oxford University Press; 2004:1212-1240.
40. Barmack NH, Baughman RW, Errico P, et al. Vestibular primary afferent projection to the cerebellum of the rabbit. J Comp Neurol. 1993;327:521-534.
41. Brodal A. The vestibular nuclei in macaque monkey. J Comp Neurol. 1984;227:252-266.
42. Voogd J, Glickstein M. The anatomy of the cerebellum. Trends Neurosci. 1998;21:370-375.
43. Langer T, Fuchs AF, Scudder CA, et al. Afferents to the flocculus of the cerebellum in the rhesus macaque as revealed by retrograde transport of horseradish peroxidase. J Comp Neurol. 1985;235:38-47.
44. Brodal A, Pompeiano O. The vestibular nuclei in cat. J Anat. 1957;91:438-454.
45. Brodal A, Pompeiano O, Walberg H. The Vestibular Nuclei and Their Connections, Anatomy and Functional Correlations. Edinburgh, Scotland: Oliver & Boyd; 1962.
46. Highstein SM, Holstein GR. The anatomy of the vestibular nuclei. Prog Brain Res. 2006;151:157-203.
47. Pompeiano O, Brodal A. The origin of vestibulospinal fibres in the cat: an experimental study, with comments on the descending medial longitudinal fasciculus. Arch Ital Biol. 1957;95:166-195.
48. Epema AH, Gerrits NM, Voogd J. Commissural and intrinsic connections of the vestibular nuclei in the rabbit: a retrograde labeling study. Exp Brain Res. 1988;71:129-146.
49. Lisberger SG, Miles FA. Role of the primate vestibular nucleus in long-term adaptive plasticity of the vestibulo-ocular reflex. J Neurophysiol. 1980;43:1725-1745.
50. McCrea RA, Strassman A, Highstein SM. Anatomical and physiological characteristics of vestibular neurons mediating the vertical vestibulo-ocular reflex of the squirrel monkey. J Comp Neurol. 1987;264:571-594.
51. McCrea RA, Strassman A, May E, et al. Anatomical and physiological characteristics of vestibular neurons mediating the horizontal vestibulo-ocular reflex of the squirrel monkey. J Comp Neurol. 1987;264:547-570.
52. Epema AH, Gerrits NM, Voogd J. Secondary vestibulocerebellar mossy fiber projections to the flocculus and uvulonodular lobule in the rabbit: a study using HRP and double fluorescent tracer techniques. Exp Brain Res. 1990;80:72-82.
53. Newlands SD, Perachio AA. Central projections of the vestibular nerve: a review and single fiber study in the Mongolian gerbil. Brain Res Bull. 2003;60:475-495.
54. Chubb MC, Fuchs AF, Scudder CA. Neuron activity in monkey vestibular nuclei during vertical vestibular stimulation and eye movements. J Neurophysiol. 1984;52:724-742.
55. Wilson VJ, Felpel LP. Specificity of semicircular canal input to neurons in the pigeon vestibular nuclei. J Neurophysiol. 1972;35:253-264.
56. Baker J, Goldberg J, Hermann G, et al. Optimal response planes and canal convergence in secondary neurons in vestibular nuclei of alert cats. Brain Res. 1984;294:133-137.
57. Duensing F, Schaefer KP. Die Aktivität einzelner Neurone im Bereich der Vestibulariskerne bei Horizontalbeschleunigungen unter besonderer Berücksichtigung des vestibulären Nystagmus. Arch Psychiatr Nervenkr. 1958;198:225-252.
58. Shimazu H, Precht W. Inhibition of central vestibular neurons from the contralateral labyrinth and its mediating pathway. J Neurophysiol. 1966;29:467-492.
59. Abend WK. Response to constant angular accelerations of neurons in the monkey superior vestibular nucleus. Exp Brain Res. 1978;31:459-473.
60. Ohgaki T, Curthoys IS, Markham CH, et al. HRP morphology of functionally identified vestibular type I neurons in the cat. Adv Otorhinolaryngol. 1988;41:14-19.
61. Pompeiano O, Mergner T, Corvaja N. Commissural, perihypoglossal and reticular afferent projections to the vestibular nuclei in the cat: an experimental anatomical study with the method of retrograde transport of horseradish peroxidase. Arch Ital Biol. 1978;116:130-172.
62. Igarashi M. Vestibular compensation: an overview. Acta Otolaryngol Suppl (Stockh). 1984;406:78-82.
63. Langer T, Fuchs AF, Chubb MC, et al. Floccular efferents in the rhesus monkey as revealed by autoradiography and horseradish peroxidase. J Comp Neurol. 1985;235:26-37.
64. Carpenter MB, Cowie RJ. Connections and oculomotor projections of the superior vestibular nucleus and cell group ‘y.’. Brain Res. 1985;336:265-287.
65. Waespe W, Henn V. Motion information in the vestibular nuclei of alert monkeys: visual and vestibular input vs. optomotor output. Prog Brain Res. 1979;50:683-693.
66. Cazin L, Magnin M, Lannou J. Non-cerebellar visual afferents to the vestibular nuclei involving the prepositus hypoglossal complex: an autoradiographic study in the rat. Exp Brain Res. 1982;48:309-313.
67. Leonard CS, Simpson JI, Graf W. Spatial organization of visual messages of the rabbit’s cerebellar flocculus. I. Typology of inferior olive neurons of the dorsal cap of Kooy. J Neurophysiol. 1988;60:2073-2090.
68. Kato I, Harada K, Nakamura T, et al. Role of the nucleus reticularis tegmenti pontis on visually induced eye movements. Exp Neurol. 1982;3:503-516.
69. Ito M. Cerebellar control of the vestibulo-ocular reflex around the flocculus hypothesis. Ann Rev Neurosci. 1982;5:275-296.
70. Lisberger SG, Fuchs A. Role of primate flocculus during rapid behavioral modification of the vestibulo-ocular reflex. I. Purkinje cell activity during visually guided horizontal smooth-pursuit eye movements and passive head rotation. J Neurophysiol. 1978;41:733-763.
71. Waespe W, Cohen B, Raphan T. Role of the flocculus and paraflocculus in optokinetic nystagmus and visual-vestibular interactions: effects of lesions. Exp Brain Res. 1983;50:9-33.
72. Chen-Huang C, McCrea RA. Viewing distance related sensory processing in the ascending tract of Deiters vestibulo-ocular reflex pathway. J Vestib Res. 1998;8:175-184.
73. Fuchs AF, Kimm J. Unit activity in vestibular nucleus of the alert monkey during horizontal angular acceleration and eye movement. J Neurophysiol. 1975;38:1140-1161.
74. Tomlinson RD, Robinson DA. Signals in vestibular nucleus mediating vertical eye movements in the monkey. J Neurophysiol. 1984;51:1121-1136.
75. McCrea RA, Baker R. Anatomical connections of the nucleus prepositus of the cat. J Comp Neurol. 1985;237:377-407.
76. Pompeiano O, Walberg F. Descending connections to the vestibular nuclei: an experimental study in the cat. J Comp Neurol. 1957;108:465-504.
77. Strassman A, Highstein SM, McCrea RA. Anatomy and physiology of saccadic burst neurons in the alert squirrel monkey. II. Inhibitory burst neurons. J Comp Neurol. 1986;249:358-380.
78. Stampacchia G, Manzoni D, Marchand AR, et al. Convergence of neck and macular vestibular inputs on vestibulospinal neurons projecting to the lumbosacral segments of the spinal cord. Arch Ital Biol. 1987;125:201-224.
79. Maeda M. Neck influences on the vestibulo-ocular reflex arc and the vestibulo-cerebellum. Prog Brain Res. 1979;50:551-559.
80. Magras IN, Voogd J. Distribution of the secondary vestibular fibers in the cerebellar cortex: an autoradiographic study in the cat. Acta Anat (Basel). 1985;123:51-57.
81. Voogd J. The Cerebellum of the Cat: Structure and Fibre Connexions, 1st American Edition. Philadelphia: Davis; 1964.
82. Gacek RR. Location of abducens afferent neurons in cat. Exp Neurol. 1979;64:342-353.
83. Gacek RR. Location of brain stem neurons projecting to the oculomotor nucleus in the cat. Exp Neurol. 1977;57:725-749.
84. Scudder CA, Fuchs AF. Physiological and behavioral identification of vestibular nucleus neurons mediating the horizontal vestibulo-ocular reflex in trained rhesus monkeys. J Neurophysiol. 1992;68:244-264.
85. Minor LB, McCrea RA, Goldberg JM. Collateral projections of medial vestibulospinal tract neurons to the extraocular motor nuclei in the squirrel monkey. Exp Brain Res. 1990;83:9-21.
86. Wilson VJ, Peterson BW. Peripheral and central substrates of vestibulospinal reflexes. Physiol Rev. 1978;58:80-105.
87. Asanuma C, Thach WT, Jones EG. Distribution of cerebellar terminations and their relation to other afferent terminations in the ventral lateral thalamic region of the monkey. Brain Res. 1983;286:237-265.
88. Kotchabhakdi N, Rinvik E, Walberg F, et al. The vestibulothalamic projections in the cat studied by retrograde axonal-transport of horseradish-peroxidase. Exp Brain Res. 1980;40:405-418.
89. Meng H, May PJ, Dickman JD, et al. Vestibular signals in primate thalamus: properties and origins. J Neurosci. 2007;27:13590-13602.
90. Horowitz SS, Blanchard JH, Morin LP. Intergeniculate leaflet and ventral lateral geniculate nucleus afferent connections: an anatomical substrate for functional input from the vestibulo-visuomotor system. J Comp Neurol. 2004;474:227-245.